Abstract
Traditional wet rendering leads to the degradation of polyunsaturated fatty acids in fish oil. Therefore, we combined this method with high-shear homogenization and high-frequency ultrasound to extract oil from Clarias magur visceral biomass. This way, we aimed to achieve higher oil yield, shorter extraction times, and a better preservation of polyunsaturated fatty acids.High-shear homogenization and high-frequency ultrasound increased the oil yields by 9.17 and 10.55%, respectively, compared to traditional wet rendering. The oil quality was also improved, with lower acid and peroxide values. Scanning electron microscopy confirmed enhanced cell disruption for increasing the oil extraction efficiency. Fourier transfer infrared spectroscopy also proved the efficacy of homogenization and ultrasound pretreatment in enhancing the extraction of polyunsaturated fatty acids from C. magur visceral biomass. Their content showed a significant variation among different extraction methods. Specifically, the high-frequency ultrasound method resulted in a notable 15.1% increase, while the high-shear homogenization method demonstrated a significant 13.3% increase, compared to the wet rendering method (control). The oil extracted by the high-frequency ultrasound method demonstrated a 7.5% increase in eicosatetraenoic acid and a 11.7% increase in docosahexaenoic acid, as compared to the oil obtained from the control method. High-shear homogenization and high-frequency ultrasound shortened the extraction time and reduced the temperature requirements for oil extraction from wet biomass.
These techniques have potential for efficient fish oil extraction, valuable in the healthcare and food industries.
Keywords
Catfish waste management, wet rendering, high-shear homogenization, high-frequency ultrasound, polyunsaturated fatty acids, lipidsINTRODUCTION
Fish oil, a key source of polyunsaturated fatty acids (PUFAs), is becoming more popular due to its health benefits. The fish oil market was worth USD 2.5 billion in 2020, and it is predicted to grow to USD 3.3 billion by 2025, with a 5.8% growth rate per year [1]. The demand for fish oil is increasing due to its wide applications in health supplements, medicines, and animal feed. PUFAs in fish oil can improve heart health and brain function, reduce inflammation, and even prevent cancer [2].
Catfish is one of the most widely farmed fish species globally, with a high production rate and low production costs, making it an affordable source of protein [3]. The viscera of catfish, including the liver and gut, are usually considered as waste and therefore discarded. However, catfish viscera contain significant amounts of lipids, proteins, and other bioactive compounds, making them an ideal source of PUFAs and other valuable components [4].
Traditional fish oil extraction methods, such as solvent extraction, cold pressing, enzymatic hydrolysis, and supercritical fluid extraction, have certain drawbacks, including safety concerns, low oil yield, high cost, and capital investment requirements [5]. As a result, researchers are now searching solvent-free, ecofriendly, and cost-effective alternatives. Wet rendering, a method that involves cooking fish in water or steam to release oil and separate it from other components, has emerged as a promising approach for fish oil extraction. This method offers potential advantages in terms of safety, oil yield, and affordability, making it a suitable option for recovering fish oil [6]. However, high temperature and prolonged cooking time lead to a thermal degradation of PUFAs and therefore affect the quality of fish oil.
By combining wet rendering with such methods as enzymatic treatment, ultrasonication, and high-shear homogenization, we can achieve higher oil extraction rates while minimizing thermal degradation [5]. Enzymatic treatment offers an advantage of breaking down cellular structures and releasing oils more effectively [7]. Ultrasonication, through application of high-frequency vibrations, disrupts cellular structures and enhances oil release, increasing the mass transfer rate and improving oil extraction efficiency with reduced thermal degradation [8]. Furthermore, incorporating high-shear homogenization into wet rendering significantly enhances overall oil extraction efficiency by mechanically disrupting cellular structures, improving mass transfer, and reducing the particle size [9]. This combination of methods contributes to higher oil yields with minimized thermal degradation. However, enzymatic treatment, despite its effectiveness, may have some limitations. For instance, it can exhibit limited specificity, impacting its efficiency on different types of fish tissues [10]. Moreover, enzymes require specific reaction conditions, such as pH and temperature, which can add complexity to the extraction process. Additionally, the cost associated with acquiring and using enzymes tends to be higher compared to high-frequency ultrasound and high-shear homogenization techniques [11].
In this study, we combined the wet rendering method with high-shear homogenization and high-frequency ultrasounds to extract fish oil from catfish visceral biomass. We investigated the effects of the extraction methods on the recovery/yield of oil, acid and free fatty acid values, oxidative stability, microstructure, compatibility, fat composition, and lipid profile. Our primary objectives were to enhance oil yield, reduce extraction time, and improve PUFAs preservation.
STUDY OBJECTS AND METHODS
Chemicals. Analytical-grade chemicals were utilized in this study. These include thiobarbituric acid, trichloroacetic acid, ammonium thiocyanate, ferrous chloride, and sodium hydroxide procured from Merck (Darmstadt, Germany). Solvents – chloroform, methanol, and hexane – were supplied by Lab-Scan (Bangkok, Thailand). Other chemicals like the Supelco® 37 component FAME mix, 1,1,3,3-tetramethoxypropane, and additional ammonium thiocyanate were sourced from Sigma-Aldrich (St. Louis, MO, USA). Cumene hydroperoxide and 2-thiobarbituric acid were obtained from Fluka Co. (Buchs, St. Gallen, Switzerland).
Raw material. Freshly available Clarias magur visceral biomass was procured from the Huatake fish market at Ladkrabang (Bangkok, Thailand). Approximately 2–3 kg of viscera was packed in polyethylene bags, placed into a polystyrene container with ice and transported to the School of Food Industry, King Mongkut’s Institute of Technology Ladkrabang. Upon arrival, the visceral biomass was ground using a meat grinder (Model: HR271331, Philips, Netherlands), and the ground sample was used for further analysis.
Proximate composition. The standard AOAC method numbers such as 930.15, 923.03, 920.39, and 923.01 were used to determine the moisture, protein, fat, and ash contents in fresh C. magur visceral biomass [12].
Experimental design. We applied the Taguchi orthogonal array design to assess the effect of high-shear homogenization and ultrasonication on C. magur visceral oil extraction via wet rendering. A four-factor, three-level design was used to study the impact of each variable on the oil yield, recovery, quality (acid value, free fatty acids), and oxidative stability (peroxide value, thiobarbituric acid reactive substances) [13]. The variables for high-shear homogenization-assisted extraction included the homogenization speed and time (6500– 26 000 rpm, 5–15 min), as well as the cooking temperature and time (40–80°C, 5–15 min). High-frequency ultrasound-assisted extraction included such variables as the amplitude and sonication time (40–80%, 5–15 min), as well as the aforementioned cooking conditions.
Oil extraction. High-shear homogenization-assisted extraction. Accurately weighed (100 g) ground visceral biomass was mixed with distilled water at a ratio of 1:0.5 (w/v). The mixture was subjected to a high-shear homogenization (T-25 Digital Ultra Turrax, IKA, Thailand) at different homogenization speeds and times (Table 1). The homogenized mixtures were exposed to wet rendering with various cooking temperatures and times (Table 1). The oil was separated as described by Pudtikajorn et al., with slight modifications [14]. After each experimental run, the sample temperature was allowed to be brought down to room temperature and the extraction mixture was filtered through muslin cloth and then through Whatman No. 1 filter paper. The filtrate was further centrifuged at 2500 g for 15 min, the cell debris pellets were discarded, and the liquid sample was separated into the oil and aqueous phase using a separating funnel.
The recovered oil was used for analysis, the excess oil was transferred into an amber bottle, whereas headspace was flushed using nitrogen gas and stored at –20°C.
High-frequency ultrasound-assisted extraction. The minced visceral biomass (100 g) was mixed with distilled water at a ratio of 1:0.5 (w/v). The mixture was subjected to an ultrasound (Vibra-Cell processor, Sonics & Material, Inc., Newtown, CT, USA) at different amplitudes and times, followed by wet rendering with various cooking temperatures and times (Table 2). After each experimental run, the sample was filtered, and the oil was separated, as described earlier [14]. The recovered oil was used for further analysis. Additionally, the oil obtained from the simple wet rendering process was considered as a control. The process parameters were a viscera:water ratio (w/v) of 1:0.5, extraction temperature of 90°C, and extraction time of 20 min.
Extraction yield. The oil extraction yield, g/100 g (wet basis), was calculated using the following Eq. (1) [14]:
Oil quality. Acid value. The acid value was measured using a method suggested by Chaijan et al., with slight modifications [15]. One gram of oil was dissolved in 10 volumes of hexane and titrated with 0.02 N KOH after adding two drops of phenolphthalein until the pink color was achieved. The acid value was expressed as milligrams of KOH neutralizing free acids in a gram of oil, and was calculated accordingly.
where N is the normality of KOH; Vs is the volume of KOH required for the sample; Vb is the volume of KOH required for the blank; W is the weight of the sample, g.
Free fatty acids (FFAs). The FFA, %, content in the oil sample was calculated as a percentage of oleic acid present, utilizing the following Eq. (4):
where MWoleic acid is the molecular weight of oleic acid; MWKOH is the molecular weight of KOH.
Oxidative stability of oil. Peroxide value. The peroxide value of oil was measured spectrophotometrically as described by Bruno et al., using 20 mM ferric thiocyanate as a reducing agent [16]. The standard curve was plotted using cumene hydroperoxide at concentrations of 0 to 50 ppm and the peroxide value was expressed as mg cumene equivalents/kg of the oil sample.
Thiobarbituric acid reactive substances (TBARS). The TBARS analysis was also conducted as described by Bruno et al., with minor modifications [16]. About 10 mg of oil was mixed with 2.5 mL of thiobarbituric acid, incubated at 95°C for 10 min, cooled, and then centrifuged at 3600 g for 20 min at 25°C. The absorbance of the upper layer was measured at 532 nm. The TBARS content was determined through a standard 1,1,3,3-tetraethoxypropane curve, expressed as mg MDA/kg oil.
Characterization of oil. Three oil samples were selected for characterization, namely: 1) the oil recovered by optimized high-shear homogenization-assisted extraction; 2) the oil recovered by optimized high-frequency ultrasound-assisted extraction; 3) the oil recovered by traditional wet rendering (viscera:water = 1:0.5, extraction temperature = 90°C, extraction time = 20 min), wich considered as the control.
Scanning electron microscopy. To evaluate the impact of each extraction method, the residues obtained from high-shear homogenization-assisted extraction, high-frequency ultrasound-assisted extraction, and wet rendering, were examined under a scanning electron microscope (XL30 FEI, Philips, France) at the magnification of 500×. In preparation for scanning electron microscopy, the residual material was dried at 50°C and then coated with gold using the sputter coating technique.
Fourier transfer infrared spectroscopy (FTIR). FTIR spectra were obtained using the ATR-FTIR model Equinox 55 (Bruker, Ettlingen, Germany) at a range of 400– 4000 cm–1 with a resolution rate of 4 cm–1 [17].
Fatty acid composition. Fatty acid methyl esters (FAMEs) were prepared following Moula Ali et al. [18]. The derivatized FAMEs were analyzed via gas chromatography (GC, 7890B series, Agilent) using an HP 88 capillary column and a flame ionization detector. Gas chromatography conditions followed the manufacturer’s guidelines. Peaks were identified using Supelco® standard retention time, integrated, and calibrated against the standard curve with Open LAB CDS software (Chem Station edition, Agilent Technologies, Santa Clara, CA, USA).
Lipids nutritional quality indexes. The data from the fatty acid composition analysis were used to calculate three indexes of nutritional quality: atherogenicity index (AI), thrombogenicity index (TI), and hypocholesterolemic/hypercholesterolemic fatty acid ratio (HH).
AI represents the relationship between the cumulative amounts of primary saturated fatty acids and primary unsaturated fatty acids, with the former considered pro-atherogenic and the latter, anti-atherogenic [19]. The following Eq. (5) was used to calculate the AI:
TI indicates the relationship between pro-thrombogenetic (saturated) and anti-thrombogenetic fatty acids (MUFAs, n-6, and n-3 fatty acids) [19]. It was measured using the Eq. (6).
The HH ratio is associated with cholesterol metabolism. It was determined using the Eq. (7) [20].
Statistical analysis. The experiments were performed in triplicates and the data were presented as means ± SD. Duncan’s multiple range test was used to indicate the significant difference between the mean values. Range analysis was carried out using SPSS (version 10.0.1.0, Stat-Ease) to measure the effect of individual variables and to determine their optimum level [13]. The average response (Pij) for each variable (i = 1–3) was calculated at each level (j = 1–3). The difference between the highest and lowest values of Pij was represented as Rj and calculated to determine which variable contributed most to the quality of the oil.RESULTS AND DISCUSSION
The proximate composition of Clarias magur viscera contained 54.67 ± 0.13% of moisture, 12.21 ± 0.57% proteins, 24.35 ± 0.76% fat, and 1.86 ± 0.51% ash (w/w). Similar catfish species (i.e., C. magur from Kenya) were reported to contain 38.20% fat, which was relatively higher than the content of fat in the catfish from Thailand [21]. The difference in total fat could be due to the difference in geographical distribution and fish feed [22].
Effect of high-shear homogenization on extraction yield, oil quality, and oxidative stability. We found significant (p < 0.05) effects of various independent variables under study on the extraction yield (oil yield and recovery ranging from 4.62 to 12.31 g/100 g and 19.01 to 50.65%, respectively), oil quality (acid value and free fatty acids ranging from 10.52 to 29.31 mg KOH/g of oil and 0.53 to 1.47% oleic acid, respectively), and oxidative stability (peroxide value and thiobarbituric acid reactive substances ranging from 18.10 to 39.11 mg cumene hydroperoxide equivalent/kg of oil and 0.34 to 0.52 mg MDA eq/kg of oil, respectively) (Table 1).
Range analysis was carried out to optimize the extraction variables, consistent with ANOVA. The wet rendering time was the most significant parameter affecting the extraction yield (oil yield and recovery) while the homogenization speed was the most significant factor affecting the oil quality (acid value and free fatty acids) and oxidative stability (peroxide value and thiobarbituric acid reactive substances) (Table 1). The oil yield increased as the wet rendering time increased from 5 to 10 min. This could be correlated to a higher breakdown of adipose tissues with time and the release of more fat [23]. However, the 15 min wet rendering time resulted in lowering the oil yield. This could be caused by the prolonged wet rendering process leading to the emulsification of oil with water and proteinaceous material, making the subsequent separation steps more difficult [24].
The oil quality (acid value and free fatty acids) was significantly (p < 0.05) affected by the homogenization speed. The acid value and free fatty acids increased as the homogenization speed rose from 6500 to 26 000 rpm. Azab et al. observed that the acid value of mango kernel oil increased when extracted through high-shear homogenization at 8000 rpm [25]. Due to the strong mechanical action, the oil is more exposed to oxygen, which leads to increased oxidation [9]. The resulting free radicals and reactive oxygen species can react with fatty acids in the oil, leading to an increase in the acid value. Furthermore, the increased shear forces can cause more disruption of the oil droplets, leading to a release of more free fatty acids [9].
The breaking of the oil droplets during high-shear homogenization exposes a larger surface area of the oil to the aqueous phase, promoting hydrolysis and increasing the content of free fatty acids. Additionally, the intensified shear forces can accelerate the breakdown of lipid molecules, increasing the peroxide value [26]. The higher shear force generated at increased homogenization speeds facilitates greater oxidation, resulting in higher peroxide value levels. Moreover, the shearing action causes the breakdown of lipid molecules, leading to the production of secondary oxidation products, such as malondialdehyde (MDA), which reacts with thiobarbituric acid to form thiobarbituric acid reactive substances. Therefore, higher homogenization speeds can lead to higher thiobarbituric acid reactive substances, indicating an increase in lipid oxidation.
Effect of high-frequency ultrasound on extraction yield, oil quality, and oxidative stability. Table 2 shows that the extraction yield, oil quality, and oxidative stability of the extracted oil were significantly (p < 0.05) affected by various independent variables. The oil yield and oil recovery ranged between 3.18 to 12.28 g/100 g and 13.08 to 50.41%, respectively. The acid value and free fatty acids in the extracted oil ranged between 9.35 to 26.12 mg KOH/g of oil and 0.47 to 1.31% oleic acid, respectively. The peroxide value and thiobarbituric acid reactive substances (TBARS) ranged between 14.34 to 32.22 mg cumene hydroperoxide equivalent/kg of oil and 0.30 to 0.51 mg MDA equivalent/kg of oil, respectively.
Based on the range analysis, the ultrasound amplitude and sonication time significantly (p < 0.05) influenced the extraction yield, oil quality, and oxidative stability, while the wet rendering temperature and wet rendering time showed less significant effects. Raising the ultrasound amplitude from 40 to 60% increased the oil yield and recovery, which could be attributed to an increased cavitation effect (Table 2). This effect causes a breakdown of adipose tissues over time and leads to the release of more oil, consequently improving yield and recovery. However, when the amplitude exceeded 80%, it caused the emulsification of oil with water and protein, complicating the separation and reducing the oil yield [27]. Along with the amplitude, the sonication time significantly impacted the response variables. As the sonication time increased from 5 to 15 min, both the oil yield and recovery improved. This improvement could be due to the extended exposure to ultrasound, promoting further tissue breakdown and oil release [28]. Nevertheless, similarly to the ultrasound amplitude, excessive sonication time could lead to emulsification and a subsequent decrease in yield. Zheng et al. also observed that the prolonged sonication time (30 min) resulted in the formation of an interfacial membrane over the lipids, thereby inhibiting the extraction of oil from Schizochytrium sp. [29].Regarding the oil quality indicators (acid value and free fatty acids), both the ultrasound amplitude and sonication time significantly (p < 0.05) influenced the responses. As these factors increased, both the acid value and free fatty acids increased, too. This could be attributed to the intensification of mass transfer and mixing at the molecular level induced by cavitation, which stimulated the hydrolysis of triglycerides [30]. The resulting free radicals and reactive oxygen species can react with fatty acids present in the oil, thereby increasing the acid value. Zhang et al. observed similar trends during the ultrasound-assisted extraction of peanut oil [31]. The measures of oxidative stability, including peroxide value and thiobarbituric acid reactive substances (TBARS), also increased with higher ultrasound amplitude and sonication time.
The collapse of cavitation bubbles can result in the formation of hydroxyl radicals, which are highly reactive free radicals. These radicals can interact with fatty acids in the oil, inducing peroxidation and subsequently increasing the peroxide value. Hernández-Santos et al. observed an increase in peroxide value in pumpkin oil extracted at 80 to 100% of ultrasound amplitude [32]. Moreover, the increased cavitation resulting from higher amplitude and time facilitates the breakdown of lipid molecules, leading to the production of secondary oxidation products like malondialdehyde, which reacts with thiobarbituric acid to form TBARS [33].
In contrast, the wet rendering temperature and time exhibited less significant effects on the response variables, according to the range analysis. While these factors might influence the extraction process, their impacts were not as significant as those of the ultrasound amplitude and sonication time. This suggests that while the wet rendering conditions can be optimized, the primary focus for extraction optimization should be on ultrasound parameters.
Optimization and validation of extraction conditions. Utilizing the Taguchi orthogonal array design for optimization, the extraction conditions were aimed to maximize the oil yield and retain the quality. High-shear homogenization-assisted extraction (HAE) was optimized at 13 000 rpm homogenization speed, 5 min homogenization time, 60°C wet rendering temperature, and 10 min wet rendering time. High-frequency ultrasound-assisted extraction (UAE) was optimized with 60% amplitude, 5 min sonication time, 60°C wet rendering temperature, and 10 min wet rendering time.
The experimental validation of the optimized conditions significantly increased the oil yield while preserving the quality. Specifically, the oil yield from HAE was approximately 9.17% higher (11.89 g/100 g) than that from the control method (10.90 g/100 g). Meanwhile, the UAE method yielded a remarkable increase of about 10.55% (12.05 g/100 g), showing the efficiency of ultrasound-assisted extraction. In terms of the oil quality, both the HAE and UAE methods showed substantially lower acid values compared to the control method. In particular, the acid values of 12.94 mg KOH/g oil for HAE and 14.15 mg KOH/g oil for UAE were 29.06 and 22.46% lower, respectively, than the acid value for the control method (18.24 mg KOH/g oil). The achieved acid values were within the Codex standard for fish oil (20 mg KOH/g) [34].
The peroxide value was 19.32 mg cumene hydroperoxide equivalent/kg oil for HAE and 19.83 mg cumene hydroperoxide equivalent/kg oil for UAE. These values were 23.35 and 21.36% lower, respectively, as compared to the one for the control method (25.21 mg cumene hydroperoxide equivalent/kg oil). This indicates a reduction in oxidation levels in the oil extracted by these methods.
The scanning electron microscopy images provided additional validation of the optimized extraction methods, as they revealed a considerable degree of cell disruption in the residues after both the HAE and UAE procedures. This indicates a more rapid oil extraction at lower temperatures (Fig. 1). The effect was more evident in UAE, suggesting that ultrasound pre-treatment led to better oil extraction than homogenization. This may be attributed to the sonoporation effect, where the application of ultrasound generates cavitation bubbles, leading to the formation of tiny pores in cell membranes and enhancing the oil release [35]. In contrast, the residues from the traditional wet rendering (control) showed minimal cell disruption, highlighting the effectiveness of the optimized extraction methods.
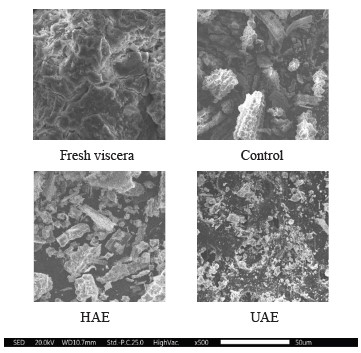
Characterization. Fourier transfer infrared (FTIR) spectra of the oil samples obtained from high-shear homogenization-assisted extraction (HAE), high-frequency ultrasound-assisted extraction (UAE), and traditional wet rendering are shown in Fig. 2. The functional group region of the spectra contained five different peaks. The peak at 3010 cm–1, which indicates the C─H stretching vibration of cis-double bonds in fatty acids, was observed at a minor intensity in the UAE and control samples (wet rendering) but was absent in the oil obtained from HAE. This difference might be due to the higher mechanical and thermal stress which may break down cis-double bonds or convert them into trans-double bonds or saturated fatty acids [36]. This process can also be increased due to higher air exposure during homogenization [9].
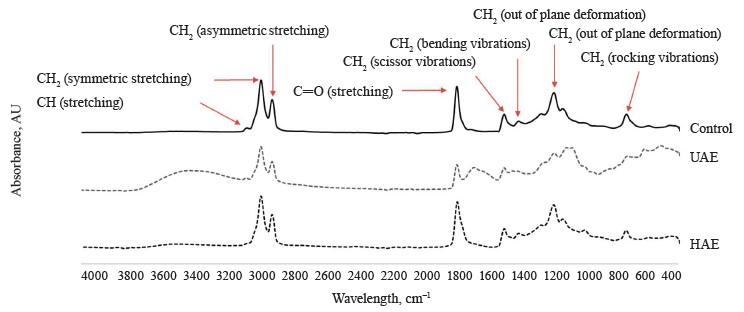
Additionally, two major peaks were observed at 2924 and 2852 cm–1, which correspond to the symmetric and asymmetric stretching vibrations of CH2, respectively. These peaks are indicative of the fatty acid chain length. Comparing these two peaks, we observed that the amplitude of the control oil sample (recovered without pretreatment) was lower than that of the oil samples obtained through HAE and UAE. The long-chain fatty acids are often tightly packed in adipose tissues, and without the mechanical disruption from homogenization or cavitation (ultrasound), these fatty acids might not be fully released, resulting in a lower intensity of the corresponding peaks in the control sample [24].
Furthermore, we identified a significant peak at 1742 cm–1, which corresponded to the C═O stretching vibration of the ester group in triacylglycerols. The peak intensity of the oil obtained through HAE and UAE was higher, suggesting a higher presence of ester bonds in triacylglycerols. In contrast, the control oil sample had a very low peak intensity. This suggests that without the pretreatment steps (homogenization and ultrasound), the adipose cells might not have been effectively ruptured to release triacylglycerols [37]. The presence of unsaturated fatty acid chains in the oil samples was indicated by the peak at 1451 cm–1, corresponding to CH2 scissor vibration. Among all the oil samples, the oil obtained through UAE had the highest amplitude, followed by the oil obtained through HAE and the control method.
The fingerprint region contained three peaks: a) CH2 bending vibration at 1362 cm–1; b) out-of-plane deformation at 1153 cm–1; and c) CH2 rocking vibration at 721 cm–1. Among these peaks, the one at 1153 cm–1 (out-of-plane deformation) corresponded to lipids and is generated due to the deformation of the C─H bonds in lipid molecules, indicating the degree of unsaturation. The oil samples recovered without pretreatment (control) had a lower amplitude compared to the oil samples recovered through UAE or HAE. This showed that the oil extracted without homogenization or ultrasound had a smaller amount of polyunsaturated fatty acids. These results were in agreement with the fatty acid composition of the oils.
Fatty acid composition. Table 3 displays the fatty acid profiles of the oils extracted using three methods: high-shear homogenization-assisted extraction (HAE), high-frequency ultrasound-assisted extraction (UAE), and traditional wet rendering process. Linoleic acid (C18:2 n-6) was the most dominant fatty acid across all three methods, with the highest concentration observed in the oil extracted by HAE (305.11 µg/g of oil). The second most abundant fatty acid was oleic acid (C18:1 n-9), with the highest level observed in the oil extracted by UAE (256.81 µg/g of oil). This finding was in agreement with Sathivel et al.
who also reported an abundance of omega-6 and omega-9 fatty acids in catfish oils. Other fatty acids were found in lower or moderate amounts [38]. We also found that the total amount of polyunsaturated fatty acids (PUFAs) was significantly higher than the total amount of saturated fatty acids (SFAs) (p < 0.05). However, eicosatetraenoic acid (EPA) and docosahexaenoic acid (DHA), long-chain omega-3 fatty acids, were present in lower concentrations across all the samples, similarly to the observations made by Sathivel et al. [38].
A notable finding was a significant influence of the extraction method on the PUFA content. The UAE method yielded the highest PUFA content (417.06 µg/g of oil), followed by HAE (410.17 µg/g of oil) and the control method (362.93 µg/g of oil) (p < 0.05). This suggests that the thermal process involved in wet rendering could negatively affect the PUFA content, as suggested by Akoh, but using an optimized extraction condition, such as UAE or HAE, can retain a considerable amount of PUFAs [39]. The oil obtained from the UAE method exhibited significantly higher amounts of EPA and DHA (29.41 and 34.91 μg/g, respectively) compared to the oil extracted from the control method (27.40 μg/g EPA and 30.80 μg/g DHA). This difference could be attributed to a higher rate of oxidation in the oil extracted via the control method, leading to the production of secondary oxidation compounds.
Lipid nutritional quality indexes. Table 3 also presents the lipid nutritional quality indices of the oils extracted using three different methods: high-shear homogenization-assisted extraction (HAE), high-frequency ultrasound-assisted extraction (UAE), and traditional wet rendering. The Omega 6:Omega 3 ratios were 3.92:1, 3.68:1, and 4:1 for the HAE, UAE, and control (wet rendering) methods, respectively. These ratios are important because they can influence inflammatory responses in the body. A lower ratio is generally preferred, as it is considered to be healthier [40]. In this case, the oil extracted through UAE had the lowest Omega 6:Omega 3 ratio, implying a better balance between these two types of fatty acids and therefore potentially healthier inflammatory responses.
Lower atherogenicity index (AI) values are desirable as they indicate lower potential for atherogenesis, a process that can lead to atherosclerosis and cardiovascular disease [41]. The oils extracted by HAE and UAE had significantly lower AI values (0.22 and 0.20, respectively) compared to the control method (0.27), indicating their lower potential for atherogenesis. The thrombogenic index (TI) assesses the potential of the oil to contribute to the formation of thrombus or blood clots [19]. A lower TI is better for heart health. Again, the oils extracted via HAE and UAE showed lower TI values (0.31 and 0.29, respectively) compared to the control method (0.38). This suggests that the oils from HAE and UAE could have a lower propensity for clot formation.
The hypocholesterolemic/hypercholesterolemic ratio (HH) indicates the balance between fatty acids that decrease low-density lipoprotein cholesterol (hypocholesterolemic) and those that raise it (hypercholesterolemic) [42]. Higher ratios are healthier as they indicate more hypocholesterolemic activity. In this study, the oils extracted via HAE and UAE had significantly higher HH values (4.29 and 4.17, respectively) compared to the control method (2.25). This suggests that the oils from the HAE and UAE methods could be healthier in terms of cholesterol balance.CONCLUSION
Our research showed that high-shear homogenization-assisted extraction and high-frequency ultrasound assisted extraction, which were optimized by using the Taguchi orthogonal array design, provide significantly improved Clarias magur oil extraction efficiency, quality, and oxidative stability compared to the traditional wet rendering method. The optimal conditions allo wed more efficient extraction of high-quality, PUFA-rich oil from C. magur viscera at lower temperatures. Analytical methods, including Fourier transfer infrared spectroscopy, fatty acid analysis, and nutritional quality indexes confirmed the superior quality of the obtained oil.
As indicated by scanning electron microscopy images, extensive cell disruption was observed in the high-shear homogenization and high-frequency ultrasound extract residues. These findings underscore the value of these methods as faster, more efficient, and low-temperature alternatives to the traditional extraction methods. Further research could expand on these findings by exploring other potential applications and scalability of these extraction methods. Our study provides promising pathways for the valuable use of catfish visceral biomass, contributing to waste minimization and enhancing the overall value chain in the fish industry.
Contribution
Jaydeep Dave conducted research activities and investigations, as well as wrote some manuscript sections. Nishant Kumar, Ashutosh Upadhyay, and Sampatee Sanguanpuag provided critical inputs during the work. Daniel Tua Purba investigated some parts of the experiments. Pikunthong Nukthamna was involved in the sample collection and wrote some sections of the manuscript. Ali Muhammed Moula Ali devised the work plan and designed the experiments, corrected and reviewed the manuscript. Sri Charan Bindu Bavisetty conducted and documented some lab experiments and contributed to the manuscript.
CONFLICTS OF INTEREST
The authors declared no conflict of interest.
ACKNOWLEDGEMENTS
A.M. Moula Ali and S.C.B. Bavisetty would like to acknowledge the support provided by the School of Food Industry at King Mongkut’s Institute of Technology Lad- krabang (Bangkok, Thailand), the Research and Innovation Services at King Mongkut’s Institute of Technology Ladkrabang, and the National Science, Research and Innovation Fund, Thailand. This work was supported by King Mongkut’s Institute of Technology Ladkrabang. Nishant Kumar and Ashutosh Upadhyay also thank the National Institute of Food Technology Entrepreneurship and Management (Kundli, Sonepat, India).
FUNDING
This work was funded by the School of Food Industry at King Mongkut’s Institute of Technology Ladkrabang (Grants #2565-02-07-007 and #2564-02-07-003), the Research and Innovation Services at King Mongkut’s Institute of Technology Ladkrabang (Grant #KREF186318), and the National Science, Research and Innovation Fund, Thailand (Grants #RE-KRIS/FF66/25 and RE-KRIS/FF66/27).REFERENCES
- The State of world fisheries and aquaculture 2022. Towards blue transformation. Rome: FAO; 2022. 266 p. https://doi.org/10.4060/cc0461en
- Dave JP, Moula Ali AM, Bavisetty SCB. An overview on recent advances in functional properties of dietary lipids, encapsulation strategies and applications. Nutrition and Food Science. 2022;52(7):1158–1180. https://doi.org/10.1108/NFS-09-2021-0282
- Hien BTT, Diem PT, Tung LA, Huong TT, Hoang NH, Bat NK, et al. Optimizing enzymatic hydrolysis for feed production from catfish by-products. Foods and Raw Materials. 2022;10(1):19–26. https://doi.org/10.21603/2308-4057-2022-1-19-26
- Sathivel S, Prinyawiwatkul W, King JM, Grimm CC, Lloyd S. Oil production from catfish viscera. Journal of the American Oil Chemists' Society. 2003;80(4):377–382. https://doi.org/10.1007/s11746-003-0707-z
- Ivanovs K, Blumberga D. Extraction of fish oil using green extraction methods: A short review. Energy Procedia. 2017;128:477–483. https://doi.org/10.1016/j.egypro.2017.09.033
- Taati MM, Shabanpour B, Ojagh M. Investigation on fish oil extraction by enzyme extraction and wet reduction methods and quality analysis. AACL Bioflux. 2018;11(1):83–90.
- Aryee ANA, Simpson BK, Villalonga R. Lipase fraction from the viscera of grey mullet (Mugil cephalus): Isolation, partial purification and some biochemical characteristics. Enzyme and Microbial Technology. 2007;40(3):394–402. https://doi.org/10.1016/j.enzmictec.2006.07.009
- Esclapez MD, García-Pérez JV, Mulet A, Cárcel J. Ultrasound-assisted extraction of natural products. Food Engineering Reviews. 2011;3:108–120. https://doi.org/10.1007/s12393-011-9036-6
- Zhang J, Ström A, Bordes R, Alminger M, Undeland I, Abdollahi M. Radial discharge high shear homogenization and ultrasonication assisted pH-shift processing of herring co-products with antioxidant-rich materials for maximum protein yield and functionality. Food Chemistry. 2023;400:133986. https://doi.org/10.1016/j.foodchem.2022.133986
- Rai AK, Swapna HC, Bhaskar N, Halami PM, Sachindra NM. Effect of fermentation ensilaging on recovery of oil from fresh water fish viscera. Enzyme and Microbial Technology. 2010;46(1):9–13. https://doi.org/10.1016/j.enzmictec.2009.09.007
- Moula Ali AM, Sant'Ana AS, Bavisetty SCB. Sustainable preservation of cheese: Advanced technologies, physicochemical properties and sensory attributes. Trends in Food Science and Technology. 2022;129:306–326. https://doi.org/10.1016/j.tifs.2022.10.006
- Official methods of analysis. Association of Official Analytical Chemists; 2017.
- Kaur N, Aggarwal P, Kumar V, Kaur S. Influence of different extraction techniques on the extraction of phytochemicals and antioxidant activities from Syzygium cumini (jamun) pomace using Taguchi orthogonal array design: A qualitative and quantitative approach. Biomass Conversion and Biorefinery. 2022;13:14497–14509. https://doi.org/10.1007/s13399-022-02826-1
- Pudtikajorn K, Sae-leaw T, Singh A, Benjakul S. Mild heating process and antioxidant incorporation increase quality and oxidation stability of oil from skipjack tuna (Katsuwonus pelamis) eyeball. European Journal of Lipid Science and Technology. 2022;124(3):2000391. https://doi.org/10.1002/ejlt.202000391
- Chaijan M, Benjakul S, Visessanguan W, Faustman C. Changes of lipids in sardine (Sardinella gibbosa) muscle during iced storage. Food Chemistry. 2006;99(1):83–91. https://doi.org/10.1016/j.foodchem.2005.07.022
- Bruno SF, Kudre TG, Bhaskar N. Impact of pretreatment-assisted enzymatic extraction on recovery, physicochemical and rheological properties of oil from Labeo rohita head. Journal of Food Process Engineering. 2019;42(3):e12990. https://doi.org/10.1111/jfpe.12990
- Moula Ali AM, Prodpran T, Benjakul S. Effect of squalene as a glycerol substitute on morphological and barrier properties of golden carp (Probarbus Jullieni) skin gelatin film. Food Hydrocolloids. 2019;97:105201. https://doi.org/10.1016/j.foodhyd.2019.105201
- Moula Ali AM, de la Caba K, Prodpran T, Benjakul S. Quality characteristics of fried fish crackers packaged in gelatin bags: Effect of squalene and storage time. Food Hydrocolloids. 2020;99:105378. https://doi.org/10.1016/j.foodhyd.2019.105378
- Dvoretsky AG, Bichkaeva FA, Vlasova OS, Andronov SV, Dvoretsky VG. Fatty acid composition of northern pike from an Arctic river (Northeastern Siberia, Russia). Foods. 2023;12(4):764. https://doi.org/10.3390/foods12040764
- Murzina SA, Voronin VP, Ruokolainen TR, Artemenkov DV, Orlov AM. Comparative analysis of lipids and fatty acids in beaked redfish Sebastes mentella Travin, 1951 collected in wild and in commercial products. Journal of Marine Science and Engineering. 2022;10(1):59. https://doi.org/10.3390/jmse10010059
- Jimoh WA, Ayeloja AA, Rasak IA, Akintoye EA, Abubakar A, Olaifa JB. Responses by African catfish (Clarias gariepinus) fed diets containing fish visceral meal as fishmeal replacer. Aquaculture Research. 2021;52(2):810–821. https://doi.org/10.1111/are.14936
- Murthy LN, Phadke GG, Unnikrishnan P, Annamalai J, Joshy CG, Zynudheen AA, et al. Valorization of fish viscera for crude proteases production and its use in bioactive protein hydrolysate preparation. Waste and Biomass Valorization. 2018;9:1735–1746. https://doi.org/10.1007/s12649-017-9962-5
- Kudre TG, Bhaskar N, Sakhare PZ. Optimization and characterization of biodiesel production from rohu (Labeo rohita) processing waste. Renewable Energy. 2017;113:1408–1418. https://doi.org/10.1016/j.renene.2017.06.101
- Moula Ali AM, Bavisetty SCB, Prodpran T, Benjakul S. Squalene from fish livers extracted by ultrasound-assisted direct in situ saponification: Purification and molecular characteristics. Journal of the American Oil Chemists' Society. 2019;96(9):1059–1071. https://doi.org/10.1002/aocs.12262
- Azab DE-SH, Almoselhy RIM, Mahmoud MH. Improving the quality characteristics of low fat toffee by using mango kernel fat, pectin, and high-speed homogenizer. Journal of Food Processing and Preservation. 2022;46(12):e17235. https://doi.org/10.1111/jfpp.17235
- Ashokkumar M, Bhaskaracharya R, Kentish S, Lee J, Palmer M, Zisu B. The ultrasonic processing of dairy products – An overview. Dairy Science and Technology. 2010;90:147–168. https://doi.org/10.1051/dst/2009044
- Ai M, Zhang Z, Fan H, Cao Y, Jiang A. High-intensity ultrasound together with heat treatment improves the oil-in-water emulsion stability of egg white protein peptides. Food Hydrocolloids. 2021;111:106256. https://doi.org/10.1016/j.foodhyd.2020.106256
- Amirante R, Distaso E, Tamburrano P, Paduano A, Pettinicchio D, Clodoveo ML. Acoustic cavitation by means ultrasounds in the extra virgin olive oil extraction process. Energy Procedia. 2017;126:82–90. https://doi.org/10.1016/j.egypro.2017.08.065
- Zheng X, Juan M, Kou X, Gao X, Liu J, Li S, et al. Investigation on the emulsification mechanism in aqueous enzymatic extraction of edible oil from Schizochytrium sp. Journal of the Science of Food and Agriculture. 2023;103(6):2904–2913. https://doi.org/10.1002/jsfa.12471
- Askarniya Z, Sun X, Wang Z, Boczkaj G. Cavitation-based technologies for pretreatment and processing of food wastes: Major applications and mechanisms – A review. Chemical Engineering Journal. 2023;454:140388. https://doi.org/10.1016/j.cej.2022.140388
- Zhang L, Zhou C, Wang B, Yagoub AE-GA, Ma H, Zhang X, et al. Study of ultrasonic cavitation during extraction of the peanut oil at varying frequencies. Ultrasonics Sonochemistry. 2017;37:106–113. https://doi.org/10.1016/j.ultsonch.2016.12.034
- Hernández-Santos B, Rodríguez-Miranda J, Herman-Lara E, Torruco-Uco JG, Carmona-García R, Juárez-Barrientos JM, et al. Effect of oil extraction assisted by ultrasound on the physicochemical properties and fatty acid profile of pumpkin seed oil (Cucurbita pepo). Ultrasonics Sonochemistry. 2016;31:429–436. https://doi.org/10.1016/j.ultsonch.2016.01.029
- Sinthusamran S, Benjakul S, Kijroongrojana K, Prodpran T, Agustini TW. Yield and chemical composition of lipids extracted from solid residues of protein hydrolysis of Pacific white shrimp cephalothorax using ultrasound-assisted extraction. Food Bioscience. 2018;26:169–176. https://doi.org/10.1016/j.fbio.2018.10.009
- Standard for fish oils, CXS 329-2017. Rome: FAO; 2017.
- Firouz MS, Sardari H, Chamgordani PA, Behjati M. Power ultrasound in the meat industry (freezing, cooking and fermentation): Mechanisms, advances and challenges. Ultrasonics Sonochemistry. 2022;86:106027. https://doi.org/10.1016/j.ultsonch.2022.106027
- Sato K. Introduction: Relationships of structures, properties, and functionality. In: Sato K, editor. Crystallization of lipids: Fundamentals and applications in food, cosmetics, and pharmaceuticals. John Wiley & Sons; 2018. pp. 1–15.
- Sathivel S, Prinyawiwatkul W, Negulescu II, King JM. Determination of melting points, specific heat capacity and enthalpy of catfish visceral oil during the purification process. Journal of the American Oil Chemists' Society. 2008;85(3):291–296. https://doi.org/10.1007/s11746-007-1191-9
- Sathivel S, Yin H, Prinyawiwatkul W, King JM. Comparisons of chemical and physical properties of catfish oils prepared from different extracting processes. Journal of Food Science. 2009;74(2):E70–E76. https://doi.org/10.1111/j.1750-3841.2009.01050.x
- Akoh CC. Food lipids. Chemistry, nutrition, and biotechnology. Boca Raton: CRC Press; 2017. 1047 p. https://doi.org/10.1201/9781315151854
- Food labeling: Nutrient Content claims; alpha-linolenic acid, eicosapentaenoic acid, and docosahexaenoic acid omega-3 fatty acids; guidance for industry small entity compliance guide. U.S. Department of Health and Human Services, Food and Drug Administration; 2016.
- Walldius G, Jungner I. Apolipoprotein B and apolipoprotein A-I: risk indicators of coronary heart disease and targets for lipid-modifying therapy. Journal of Internal Medicine. 2004;255(2):188–205. https://doi.org/10.1046/j.1365-2796.2003.01276.x
- Feddern V, Kupski L, Cipolatti EP, Giacobbo G, Mendes GL, Badiale-Furlong E, et al. Physico-chemical composition, fractionated glycerides and fatty acid profile of chicken skin fat. European Journal of Lipid Science and Technology. 2010;112(11):1277–1284. https://doi.org/10.1002/ejlt.201000072