Abstract
Livni is one of the Russian local pig breeds. We previously reported that this breed was more distinct from Duroc breed than from Landrace and the Large White breeds, which participated in the Livni breed creation. The aim of the study was to determine the SNP-based genetic signatures in fat-type Livni breed shared with commercial Landrace and the Large White breeds, and ones that are affected by putative selection.The genome-wide SNP genotyping was carried out using the Porcine GGP HD BeadChip, which contains ~ 80 000 SNPs.
Obtained breed relationship and admixture results indicated the insignificant participation of the Landrace and the Large White breeds in the formation of the modern allelofund of Livni pigs. 238 candidate genes were found in the genomic regions with selection signatures, 182 genes with described functions were identified. In the Livni and Landrace breeds, 35 common genes were detected which formed one cluster with enrichment coefficient = 4.94 and predominant HOXD genes. In the Livni and Large White breeds, the largest amounts of common genes were detected (62 in average), which formed two clusters. Cluster 1, with enrichment coefficient = 2.11, was characterized with genes involved in glucose metabolism. Cluster 2, with enrichment coefficient = 1.60, demonstrated helicase genes. Annotated clusters were not determined for the Livni breed. However, 50 candidate genes were specific to Livni pigs and associated with various growth, carcass and reproductive traits, essential for thermoregulation.
Results revealed common SNP-based genetic signatures and breeding effects in indigenous Livni compared with Landrace and Large White breeds.
Keywords
Livni breed, animal genetic resources, SNPs, pig, carcass, traitsINTRODUCTION
The pig is a major livestock species, and the global pork production primarily relies on the use of a limited number of international commercial breeds, specifically Duroc, Large White, and Landrace [1]. Intensive implementation of commercial hybrid breeds characterized by high production standards led to an impoverishment of genetic resources which in the past had a fair distribution [2]. However, recently a strong attention has been attracted to local breeds for improving genetic diversity and conservation of genetic resources. Local breeds are valued not only by adaptive traits, but also by the unique functional characteristics and intensively studied in Asia, Europe, Africa, as well as North and Latin America [3–14].
Twenty-two local breeds were recorded in the Soviet Union in 1980, which were generated by crossing of native breeds adapted to the local climate and having appropriate constitution and disease resistance with highly-productive improved European breeds [15, 16]. As a result of the interbreeding of the imported breeds and crossing them with the native animals, many pig breeds were created during 1920–1990. For example, Ukranian White Steppe was created in Askania Nova and approved in 1932; North Siberian – in Novosibirsk and approved in 1942, Urzgum – in the Kirov region and approved in 1957 – by crossing native pigs with the Large White boars. Breeds, as Kemerovo (approved in 1961), Breitov (approved in 1948), Latvian White and Lithuanian White (both approved in 1967), Semirechensk (1978), Mirgorod (1940), Tsivilsk (a cross of native Chuvash pigs with Large White boars, the breed is not approved), Mangalitsa, Altai (approved in 2015), and others were created by multiple crossbreeding procedures. [15]. According to the Department of Livestock and Breeding of the Ministry of Agriculture of the Russian Federation data, 98% of the total pig purebred population in 2020 included four breeds – Large White (66%), Landrace (15%), Yorkshire (13%) and Duroc (4%) [17]. Other breeds’ share was about 2%. Pavlova et al. consider 0.56% of the total pig population in the RF are of the local breeds – Livni, Altai, Tsivilsk, as of January 1, 2022. Four breeds make 99.46% of the RF pig herd, namely 56.9% Large White, 18.52% – Yorkshire, 18.18% – Landrace, and 5.83% – Duroc breed [18]. The dramatically reduction of local pig breeds during last 30 years finally led to remaining only Livni, Altai Meat-type, Short-Eared White, and Tsivislk. The authentic Kemerovo breed has also been mentioned for a number of years. However, according to the Yearbook on breeding work in pig husbandry in establishment of the Russian Federation for 2021, the last time a breeding farm certificate for the Kemerovo purebred was issued in 2019 [19]. It should be noted, that the certificate for the Tsivislk breed was last issued in 2021.
Livni is one of the Russian local pig breeds approved in 1949. Pigs of the Livni breed are large, white, black-mottled, black and red. At present, only a small population of Livni pigs is kept in a single farm in the Oryol region [20]. According to the Yearbook on breeding work, in pig husbandry in establishment of the Russian Federation for 2021 one certificate is issued annually for the Livni purebred, but the total number of the Livni pigs is steadily declining. At the beginning of 2022, 547 heads were purebreds, including 348 sows with the share in the total livestock of 0.24% [19]. For comparison, in 1949 the Livni livestock was 6757 purebreds (1334 sows), while in 1980 it was 27 200 purebreds (5500 sows) [21]. It is noteworthy that at the age of 6 months, Livni correspond to the bacon (meat) type. Then the active accumulation of fat begins and at the age of 10 months Livni pigs are already belong to meat-and-fat type, and with further fattening lead to fat type [21].
We previously reported that Livni breed is characterized the highest level of genetic diversity compared with commercial breeds. The neighbor-joining tree showed that this breed was the most distinct from Duroc breeds, but formed the knot bounding the branches corresponding to the Landrace and the Large White breeds. This observation confirmed the participation of these two breeds in the Livni breed creation. The aim of our study was to determine the SNP-based genetic signatures in Livni breed common with Landrace and the Large White breeds, and ones that are affected by putative selection in the genome of Livni breed and could be associated with fatty tissue formation and breed specificity.
STUDY OBJECTS AND METHODS
Samples and genotyping. For the study, we used samples (ear tissue) of Livni pigs (n = 35). Only purebred animals registered in Russian swine herdbook were selected, the origin of which is confirmed by both the pedigree data and DNA analysis. For genotyping, we selected the most unrelated individuals. Samples of all breeds were sent to the Ernst Federal Research Center for Animal Husbandry. A parentage and breed assignment of those breeds were confirmed based on the microsatellites in the laboratory of the Ernst Federal Research Center for Animal Husbandry, which has a certificate of 2020–2021 ISAG Pig STR Comparison Test (2020–2021) and has a special license issued by the Russian Ministry of Agriculture. Commercial breeding farms and the Ernst Federal Research Center for Animal Husbandry collaborate based on the contracts. In the contract, a clause states the consent of the owners (breeding farms) to use the samples with research purpose.
Moreover, the study did not involve any endangered or protected animal and all procedures were conducted according to the ethical guidelines of the L.K. Ernst Federal Science Center for Animal Husbandry. The Commission on the Ethics of Animal Experiments of the L.K. Ernst Federal Science Center for Animal Husbandry approved the protocol No. 6 of May 10, 2021. The ear tissues were collected by trained personnel under strict veterinary rules in accordance with the rules for conducting laboratory research (tests) in the implementation of the veterinary control (supervision) approved by Council Decision Eurasian Economic Commission № 80 (November 10, 2017).
Genomic DNA was extracted using the DNA Extran 2 kit (ZAO Sintol, Moscow, Russia) according to the manufacturer’s instructions. Concentrations of dsDNA solutions were determined using a Qubit 1.0 fluorometer (Invitrogen, Life Technologies, Waltham, Massachusetts, USA). The OD260/280 ratio was determined using NanoDrop 2000 (Thermo Fisher Scientific, Waltham, Massachusetts, USA).
The genome-wide SNP genotyping was carried out using an iScan microarray scanner (Illumina Inc., Singapore) using the Porcine GGP HD BeadChip (Illumina Inc., San Diego, CA, USA), which contains ~ 80 000 SNPs. In our study, we used all the capital equipment required for SNP genotyping by Illumina SNP arrays. The equipment belongs to the Center for Collective Use “Bioresources and Bioengineering of Agricultural Animals” of the Ernst Federal Research Center for Animal Husbandry (https://www.vij.ru/infrastruktura/ckp, accessed on 10 May 2021). The SNPs genotypes of Large White (n = 53) and Landrace (n = 50) breeds were included in the data set and obtained from Center for Collective Use “Bioresources and Bioengineering of Agricultural Animals” of the Ernst Federal Research Center for Animal Husbandry.
Quality control. Using PLINK 1.9 software, the SNP quality control was performed [22, 23]. All samples were subjected to filtering for genotyping efficiency (--mind 0.2). The SNPs genotyped in less than 90% of the samples (--geno), minor allele frequencies below 0.01 (--maf 0.01), and p-values below 10–6 for Hardy-Weinberg equilibrium were excluded from the analysis. The final data set used for analysis included 51 912 autosomal SNPs. Additional filters for linkage disequilibrium (LD) with r2 every 50kb (--indep-pairwise) were performed, amount of SNP passes LD-filtration amounted 24 861.
Genetic diversity, PCA, Neighbor-Net and Admixture. To assess the within-population genetic diversity, the observed (HO) and unbiased expected (UHE) heterozygosity, the rarefied allelic richness (АR), and the unbiased in-breeding coefficient (UFIS) were estimated using the R package, diveRsity [24]. Additionally, we computed the genomic inbreeding coefficient based on runs of homozygosity (ROH, FROH) as the ratio of the sum of the length of all ROHs per animal to the total autosomal SNP coverage; for ROH estimation, see the “Runs of Homozygosity Estimation” Section below). PCA was performed using PLINK v1.9 software. An R package, ggplot2, was used to visualize the results [25]. Pairwise FST values were calculated in the R package, diveRsity, and used for the construction of the Neighbor-Net tree in SplitsTree software (version 4.14.5) [24, 26, 27]. Admixture software (version 1.3.0) was employed for genetic admixture analysis and an R package, pophelper, was used for plotting the results [28, 29]. A crossvalidation (CV) procedure was used to calculate the number of ancestral populations (k) from one to five using Admixture software (version 1.3.0).
Selection signature analysis. Three different statistics were used for detecting the signatures of selection in the genome of pigs: the calculation of FST values for each SNP when comparing pairs of breeds, the estimation of the ROH islands, which were overlapped among different animals within each breed, and hapFLK analysis.
FST analysis. FST values for all SNPs were estimated for pairs of breeds using PLINK 1.9 [24]. Minor allele frequencies were below 5% (--maf 0.05) [30]. The top SNPs corresponding to 0.1% of FST values were used to represent a selection signature, according to Kijas et al. and Zhao et al. [31, 32].
Runs of homozygosity estimation. Runs of homozygosity were detected according to the window-free method for consecutive SNP-based detection using the R package, detectRUNS [33]. One SNP with a missing genotype and up to one possible heterozygous genotype in one run were allowed to avoid the underestimation of the number of ROHs that were longer than 8 Mb [34]. The minimum ROH length was set to 500 kb to exclude the common ROHs. To minimize false-positive results, the minimum number of SNPs was calculated as it was proposed by Lencz et al. and later modified by Purfield et al. [35, 36].
Putative ROH islands were defined as overlapping homozygous regions in analyzed individuals within each breed. A threshold of 50% (the minimum proportion of animals within the breed in which overlapping ROH were detected) was selected, as this was suggested in other studies [37, 38]. We applied the threshold of 0.1 Mb for the minimal overlapping length size and 5 SNP for minimum number in ROH island.
HapFLK analysis. In this study, a hapFLK analysis was performed to detect the selection signatures through haplotype differentiation among the studied breeds using hapFLK software (version 1.4.) [39]. The number of haplotype clusters per chromosome was calculated in fast-PHASE by using cross-validation and was set to 35 [40]. For detailed analyses, the hapFLK regions containing at least one SNP with a p-value threshold of 0.01 (-log10(p) > 2) were selected.
Identification of candidate genes. For candidate gene mining in the genomic regions under putative selection, the genomic localization of the regions as detected by three different statistics was used, i.e., the FST, ROH, and hapFLK methods. Regions that were overlapped and revealed by at least two different techniques were prioritized. Borders of these regions according to the 10.2 genome assembly were converted to genome assembly 11.1. Genes located on the selected regions were obtained from the Ensembl Genes Release 103 database based on the Sus scrofa gene sequence assembly [41].
Functional enrichment analysis. To understand the biological functions of the candidate genes, the Database for Annotation, Visualization, and Integrated Discovery (DAVID) was used for enrichment analysis [42]. Significant annotation clusters of enriched Kyoto Encyclopedia of Genes and Genomes (KEGG) pathways and Gene Ontology were selected using an enrichment score of more than 1.3 and a p-value of < 0.05. To learn the biological functions of annotated genes and genes not included in clusters, a comprehensive literature search including information from other species was carried out.
RESULTS AND DISCUSSION
Genetic diversity. The Livni pigs were characterized by higher level of genetic diversity assessed by the levels of observed heterozygosity, unbiased expected heterozygosity, and allelic richness as compared to the Landrace and Large White breeds. The negative value of the inbreeding coefficient UFIS indicates an excess of heterozygotes from the Hardy–Weinberg equilibrium in all the breeds (Table 1). In commercial breeds, the excess of heterozygotes was more significant compared to the Livni breed.
Breed relationship and admixture. The PCA-plot (Fig. 1a), the neighbor-joining tree (Fig. 1b) and cluster structure (Fig. 1c) showed the breed-specific distribution of individuals for all of the studied breeds. Obtained distribution indicated the insignificant participation of the Landrace and Large White breeds in the formation of the modern allelofund of Livni pigs and demonstrated that sampling is suitable for searching for loci under selection pressure in the studied pig breeds and their subsequent structural annotation.
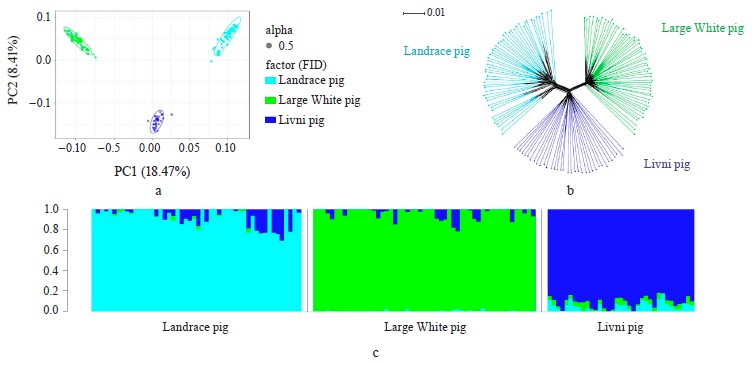
Selection signature detection. SNPs with FST-values beyond the cut-off (top 0.1%) were distributed among all autosomes, excepting SSA18. Most of these SNPs were specific to breed pairs. Six SNPs were found in SSA4 (1 SNP), SSA6 (2 SNP), and SSA11 (3 SNP), which were common for Livni – Landrace and Landrace – Large White, and seven SNPs on SSA5 (5 SNP), SSA8 (1 SNP), and SSA12 (1 SNP), for Livni – Large White and Landrace – Large White (Fig. 2).
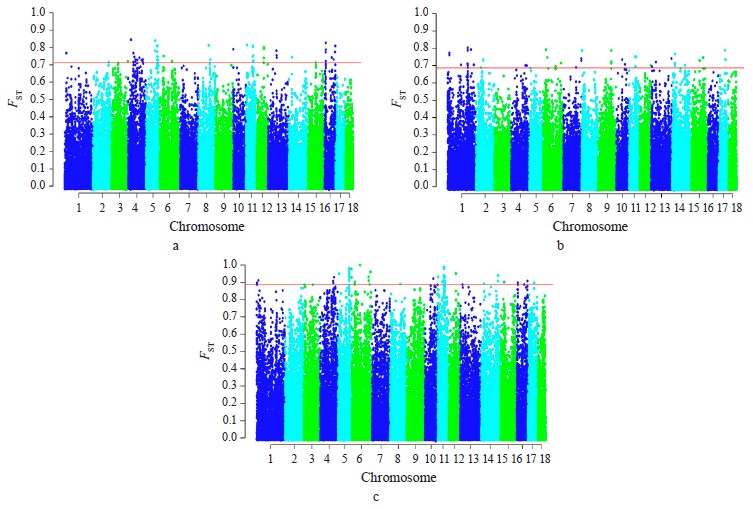
The distribution of ROH island number and length in chromosomes is presented in Table 2. Forty-two ROH islands were detected in the Livni breed, which covered 34.415 Mb of the genome, while for Landrace and Large White, 126 and 224 ROH islands covered 161.792 and 282.402 Mb of the genome, respectively. The average length of the ROH island in Livni breed was significantly lower than that of pigs of commercial breeds: 2.868 ± 0.822 Mb versus 8.988 ± 2.185 (Landrace) and 15.689 ± 2.770 Mb (Large White), respectively (p < 0.001).
Eighteen common ROH islands were detected in the Large White and Landrace breeds, which were identified in ten autosomes: SSA1 (4 ROH islands), SSA4 (3 ROH islands), SSA5, SSA6 (3 ROH islands), SSA8, SSA9, SSA11, SSA13, SSA14, SSA16, and SSA17. Eight common ROH islands were detected in the Livni and Large White breeds, which were identified in six autosomes, namely SSA1, SSA2, SSA7, SSA12, SSA14 (3 ROH islands), and SSA15. Eight common ROH islands were detected in the Livni and Landrace breeds, which were identified in five autosomes: SSA1, SSA3 (2 ROH islands), SSA4, SSA6, SSA11 (2 ROH islands), and SSA15. Five ROH islands detected in SSA1, SSA6, SSA11, SSA14, and SSA15 were common for three breeds (Table 3).
The hapFLK analysis resulted in the identification of 13 putative regions affected by the selection (Fig. 3). These regions were distributed among 10 autosomes, including regions on SSA1, SSA3, SSA14, and SSA13 with a statistical significance of p < 0.001. Four regions were Large White-specific, three – Landrace-specific, three – Large White and Landrace-specific, three – Livni and Large White-specific (Table 4).
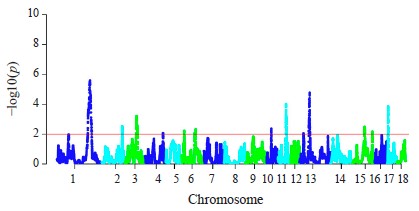
Comparing the genomic localization of the regions under putative selection detected by three different statistics (FST, ROHs, and hapFLK) revealed the presence of 13 overlapping regions, which were identified by at least two different methods (Table 5); 7 regions corresponded to the Large White breed, 2 corresponded to the Landrace breed, 2 were common to Large White and Landrace breeds, and 2 were common to Large White and Livni breeds. Additionally, in the list of genes for structural and functional annotation, we included ROH islands identified only in Livni pigs, as well as common ROH islands identified in the Livni and one or two compared breeds. Thus, 16 Livni-specific regions, and 39 regions, which are common for both two and more breeds were selected for the structural and functional annotation.
Candidate gene determination and functional enrichment determination. The structural annotation of these regions revealed the presence of 238 candidate genes: 50 genes were specific to Livni pigs, 62 to Livni and Large White pigs, 35 to Livni and Landrace pigs, 36 to all studied breeds, and 55 were specific to Large White and Landrace pigs (Table 6).
Using the DAVID web tool and a list of 238 candidate genes found in the genomic regions with selection signatures, 182 genes with described functions were identified. The significant clusters are shown in Table 7. Annotated clusters with an enrichment coefficient – log10(p) > 1.3 (corresponds to p < 0.05) were not determined for the Livni breed and all three studied breeds (Livni, Large White and Landrace). Two reliably annotated clusters were identified for the Livni and Large White, Large White and Landrace breeds, and one annotated cluster for Livni and Landrace. For the list of Livni and Large White genes, the presence of two annotated clusters was revealed. Cluster 1 (enrichment coefficient = 2.11) included G6PC2, HKDC1, HK1 genes involved in carbohydrate metabolism. Cluster 2 (enrichment coefficient = 1.60) included SUPV3L1, SLC25A16, HKDC1, DDX21, PIK3C2A, MAP3K7, DDX50, and HK1 genes involved in the processes of DNA replition and repair. For Livni and Landrace one reliable cluster (enrichment coefficient = 4.49) was determined, including the genes CIART, HORMAD1, HOXD3, HOXD4, HOXD8, HOXD9, HOXD10, HOXD12, HOXD13, EVX2, NR2E1, and PLEKHO1. Genes under selection pressure in commercial pig breeds (Large White and Landrace) were combined into two reliable clusters. Cluster 1 (enrichment co-efficient = 1.74) combined the genes KCNA1, KCNA6, KV1.5, and SLC30A9 involved in the regulation of ion transmembrane transport, mainly potassium. The IBTK, KCNA1, KCNA6, and ZBTB10 genes regulating transcription repression and interaction with components of histone deacetylase co-repressor complexes were localized in cluster 2 (enrichment coefficient = 1.54).
Specific and overlapping sites in the genome of Livni, Large White and Landrace breeds that are under selection pressure have been identified. Positional candidate genes were identified and their annotation was performed. In the current study, three pig breeds were examined and compared. We previously reported that Livni breed is characterized the highest level of genetic diversity compared with commercial breeds. The neighbor-joining tree showed that this breed was the most distinct from Duroc but formed the knot bounding the branches corresponding to the Landrace and the Large White breeds. This observation confirmed the participation of these two breeds in the formation of the Livni breed during it artificial selection. We observed the highest level of genetic diversity in Livni pigs compared to commercial breeds (Table 1), which may be a consequence of the participation of various breeds in the development of the Livni breed, including Large White and Landrace. However, results of breed relationship and admixture revealed distribution indicated the insignificant participation of the Landrace and Large White breeds in the formation of the modern allelofund of the Livni pigs.
Using three different statistics (top 0.1 FST at pairwise breed comparison, ROH islands and hapFLK analysis), we selected 13 overlapping regions, which were identified by at least two different methods (Table 2); 7 regions corresponded to the Large White breed, 2 corresponded to the Landrace breed, 2 were common to Large White and Landrace breeds, and 2 were common to Large White and Livni breeds. Among 238 candidate genes, which were localized within selected genomic regions (Table 3), 182 genes had the described functions in GO-terms; among them, 50 genes were specific to Livni pigs, 62 were specific to Livni and Large White pigs, 35 were specific to Livni and Landrace pigs, 36 were specific to all studied breeds, and 55 were specific to Large White and Landrace pigs (Table 3).
Among common genes for three studied breeds, MLANA and JAK2 were previously observed in Livni and Duroc breeds and involved in adipogenesis [20, 43]. It was reported that FKBP7 is highly expressed in subcutaneous adipose tissue of mature Erhualian pig, while CHAT is essential for macrophages as a source of acetylcholine for the regulation of adaptive thermogenesis [44, 45]. HSPH1 is a known marker of both human and mouse brown adipocytes and was upregulated in young and old brown adipocytes after acute cold exposure [46]. HSPA1L were found to be differently expressed between the low and high drip loss groups in the Duroc pigs [47]. NCOA4 may play a role in early events of adipocyte differentiation and were found in Pudong White pigs [48, 49]. PLGRKT coordinately regulates multiple aspects of adipose function and was found to be related to obesity [50, 51]. According to Gene Ontology terms, ALOX5 is strongly associated with immunity, lipid metabolism and fat cell differentiation, insulin secretion, and oxidative stress. Interestingly, this gene was also very highly significantly associated with feet and leg structure soundness traits in pigs [52]. OSBPL6 linked with lipid and sterol transport and encoded by miR-33, which may also regulate adaptive thermogenesis [53]. PLEKHA3 is also associated with lipid metabolism, and mutations were identified for this gene in the Puławska pig breed, which is characterized by thicker backfat and better meat quality values [54]. PARG is linked with carbohydrate metabolic process and could be involved in lipid metabolism [55]. According to Gene Ontology terms, ERMP1 involved incellular response to oxidative stress, HEYL – in skeletal muscle cell differentiation. INSL6 was linked with male fertility in Enshi pigs and reproduction in Anhui pigs [56, 57]. MSMB was closely related to body weight, body height, abdominal circumference, and chest depth in Xiangsu hybrid pigs [58]. OGDHL was up-regulated in the liver in pigs with higher backfat thickness of Songliao black female pig population [59]. Although PRKRA is strongly associated with immune response, including piglets, this gene plays unexpected role in the regulation of mitochondrial biogenesis and energetics in cells and brown adipocytes [60, 61]. ZFAND4 gene encodes stress proteins and was detected in Pudong White pigs, as well as ZNF22 [49, 62]. C10orf10 is involved in adipose tissue thermogenesis and was observed in heavy Iberian Pigs [63, 64]. PDL1 was determined as candidate biomarkers for predicting residual feed intake in Yorkshire pigs, as well as U1 [65, 66]. RLN is a candidate gene for reproductive traits in pigs and was found to regulate adipose tissue development through stimulating adipogenesis and modulating adipocyte metabolism [67–69]. SNORA19 could be involved in body temperature regulation [70]. U6 was associated with litter traits in Yorkshire and Landrace pigs and was a selection signature gene in Meishan population [71, 72].
In the Livni and Landrace breeds, 35 common genes were detected, which formed one cluster with enrichment coefficient = 4.94 and predominant HOXD genes. According to Gene Ontology terms, HOXD10 and HOXD9 are involved in various developmental processes, such as single fertilization, skeletal muscle tissue development, adult locomotory behavior, embryonic skeletal system morphogenesis, peripheral nervous system neuron development, neuromuscular process, etc. HOXD10 is required systemically for secretory activation in lactation [73]. Expression level of HOXD10 was increased in animals with high marbling [74]. HOXD9 and HOXD10 are associated with such traits as growth, body weight and composition, abdominal fat, organogenesis, and feed intake and consumption [75]. They also play an active role in chondrogenesis and the development of adipose depots [76, 77]. HOXD3, HOXD8, HOXD12, and HOXD13 are also associated with skeletal system development. HOXD12 is differently expressed between large and small piglet size [78]. HOXD3 is also associated with nervous system development, considered as predictors for feed efficiency traits [79, 80]. It was reported that HOXD4 and HOXD8 are up-regulated in differentiated adipocytes [81]. HOXD8 gene is involved in patterning the lower thoracic and lumbar vertebrae, in the urogenital tract development, also of mesoderm origin [82, 83]. HTR6, associated with nervous system, was identified as interesting candidate genes involved in axonogenesis and synapsis in Iberian breed [84]. ADAMTSL4 was found to evolve under positive selection and exhibited significant down-regulated mRNA expression in the Tibetan pigs [85]. ABCC1 is expressed in adipose and skeletal muscle, up-regulated in obesity, and involved in the embryo development of pig; it was also detected in Northeast wild boar [86–89]. HORMAD1 is linked with embryo development and productivity. Z. Zeng et al. noted HORMAD1 to belong to growth-related Meishan pig genes [90]. HORMAD1 was under heavy selection based on runs of homozygosity in a Large White pig population and associated with obesity [91]. SEC63 was determined as candidate genes for estimated breeding values feed conversion ratio in Maxgro boars [92]. It was found an association between the CIART genotype and backfat thickness in Duroc pigs, and its expression is affected by food intake [93, 94]. According to Gene Ontology terms, ENSA is associated with regulation of insulin secretion and related to adipocyte development [95]. ECM1 is involved in immunity and bone development. It was reported to be an important gene highly expressed in subcutaneous white adipose tissue (sWAT) as compared to brown adipocytes, and was determined in Korean Wild Boar, up-regulated in Congjiang Xiang pigs with large litter size and in testis tissue from Duroc boars [96–99]. KLHL1 could be linked with Landrace and Yorkshire pig backfat thickness in Korea and involved in environmental adaptation [100, 101]. NR2E1 is involved in developmental processes and linked with environmental adaptation concerning behavioral defense response in Xiang pigs [102]. It showed significant associations with feed conversion efficiency and growth rate in pigs [92]. PRPF3 gene is differentially expressed in the Longissimus dorsi muscle being more abundant in Large White than in Wujin pigs [103]. VPS45 could be linked with growth trait [104]. FOXO3A promotes metabolic adaptation and stress resistance in hypoxia, associated with carcass length, backfat thickness and drip loss, related to muscle development in Iberian pigs [105–107]. FOXO3A could promote lipid accumulation as well [108]. Ssc-mir-10b was downregulated in Tibetan pigs, related to hypoxia adaptation, play important roles in fat-related processes in adipose tissue, had been frequently reported highly expressed in skeletal muscle during porcine prenatal and postnatal developmental stages and abundantly expressed in subcutaneous adipose tissue in pigs [109–113].
In the Livni and Large White breeds the largest amount of common genes was detected and averaged 62, which formed two clusters. Cluster 1, with enrichment coefficient = 2.1, was characterized with genes involved in glucose metabolism. Among them, G6PC2, HKDC1 and HK1 are critical for glucose homeostasis. HK1 effects on growth and meat quality in Polish Landrace [114]. It is important for sperm motility in Duroc, enriched in brown adipocytes of aged mice, up-regulated by severe cold and essential for brown adipocytes thermogenesis [115–118]. Cluster 2, with enrichment coefficient = 1.60, demonstrated helicase genes. DDX21 is associated with immunity and belongs to the top 4 lymphocyte associated genes in pigs [119]. SUPV3L1 is important for the maintenance of the skin barrier and related to percentage of certain fiber types [120, 121]. MAP3K7 is also linked with immunity and strongly associated with neuropsychiatric processes [122]. It was reported to be associated with growth traits and adipocyte differentiation [100, 123]. PIK3C2A gene is related to hepatic insulin resistance and steatosis, average daily gain and lean meat percentage, intramuscular fat and backfat thickness in two Duroc populations, being under positive selection in all high-altitude species [124–127]. According to Gene Ontology terms, ABCB11 is associated with fatty and bile acid metabolic process and could be involved in gene networks for intramuscular fatty acid composition in porcine [128, 129]. ABCC8 was reported to be selection region for intramuscular fat and backfat thickness in two Duroc populations, and the most down-regulated genes in the group with higher backfat thickness in Yimeng black pigs [126, 130]. DEF6 is linked with average backfat thickness [131]. FKBP5 is associated with immunity, backfat thickness and leaf fat weight, significantly contributed to residual feed intake [79, 131–133]. Expression of this gene is inversely associated with the expression of lipolytic, lipogenic and adipogenic genes [134]. According to Gene Ontology terms, LDB3 is associated with heart development and muscle structure development, related to muscle growth traits in pig and may have potential roles in environmental adaptation [135, 136]. ARMC12 regulates spatiotemporal mitochondrial dynamics during spermiogenesis and is required for male fertility [137]. BMPR1A is associated with numerous developmental processes, identified as a novel candidate gene affecting the number of thoracic vertebrae in pigs, and regulates the development of hypothalamic circuits that are critical to the feeding behavior [138, 139]. Additionally, BMPR1A is important in brown fat development and involved in browning of white adipose tissue [140, 141]. CCAR1 positively regulates adipocyte differentiation [142]. CLPSL2 and CLPS are linked with digestion, lipid catabolic process, and response to food. CLPSL2 could be involved in the regulation of acrosomal integrity, spermatozoa motility, and male fertility, while CLPS demonstrated effect on some characteristics connected with lean content of the carcass and fat content and affected intramuscular fat content [143–145]. It may be associated with former selection toward reduced fat content in carcass [114]. According to Gene Ontology terms, COL13A1 is associated with skeletal system development, was under significant positive selection Yorkshire pigs and associated with fat deposition, as well as HNRNPH3 [146–148]. JMJD1C is potentially associated with cold adaptation [149, 150]. It demonstrates the positive selection in regulation of various reproductive traits in pigs [151–154]. JMJD1C was identified in Tibetan pigs that are well adapted to the high altitude [155]. On the other hand, this gene have been associated with white blood cells in Large White pigs, identified as a novel regulator of adipogenesis and contributed to browning [156–158]. According to Gene Ontology terms, MYOD1 and MYPN are strongly involved in skeletal muscle tissue development. It was reported about potential role of MYOD1 in body-fat distribution regulation [159]. Mutations in the MYOD1 gene show a significant effect on the pork meat quality and single nucleotide polymorphisms in the porcine MYOD1 affected on meat quality traits and carcass traits in heavy pigs [160–162]. MYPN is related to body composition and can be considered as candidate for meat and carcass traits in pigs [163–165]. NRBF2 is linked with energy metabolism and was specific selective for Tibetan pig [155]. NUCB2 is expressed in fat depots of the pig and that level of expression is sensitive to stimulation of appetiteregulating pathways in the hypothalamus [166]. It plays an important role in whole-body energy homeostasis and body weight at puberty by regulation of appetite of Jinhua Pigs [126]. NUCB2 is also involved in cold adaptation, indicating that central nesfatin-1 regulates thermogenesis [167, 168]. REEP3 mediates adipogenic differentiation [169]. According to Gene Ontology terms, SIRT1 is linked with regulation of lipid storage, white and brown fat cell differentiation, adipose tissue development, etc. It is implied in the browning of white adipose tissue, promotes lipid metabolism and mitochondrial biogenesis in adipocytes and coordinates abiogenesis by targeting key enzymatic pathways [170, 171]. Apart from that, it negatively correlates with intramuscular fat content and demonstrates protective role in skeletal muscle’s adaptation to cold stress [172, 173]. SNCG controls metabolic functions in fat cells and belongs to white adipose tissue-selective genes [174, 175]. ZNF76 is very close to peroxisome proliferative activated receptor delta (PPARD) at 35 Mb, which is a positional and physiological candidate for affecting backfat thickness [176]. RNF213 is involved in adipogenesis and emerged as a link between obesity, inflammation, and insulin resistance [177, 178]. SNORD14 were more expressed in Large White heavy pigs with high intramuscular fat content [179]. U3 was identified as a promising candidate gene for average backfat thickness in multiple pig breeds and populations [180].
Annotated clusters with an enrichment coefficient –log10(p) > 1.3 (corresponds to p < 0.05) were not determined for the Livni breed. However, 50 candidate genes were specific to Livni pigs. DLGAP5 is a stillbirth associated gene involved in lipid deposition-related pathways and significantly associated with intramuscular fat content [181–183]. ERCC4 is also associated with intramuscular fat content, presented in Tibetan wild boar and related to “response to UV” [151, 184, 185]. GPR63 has been identified as a receptor for intercellular lipid messengers and associated with reproduction traits [186, 187]. According to Gene Ontology terms, LDB3 is involved in heart and muscle structure development, while PBX3 – in various important developmental processes. PDZRN3 and ATG14 could affect intramuscular fat content in Suhuai pigs [183, 184]. They are involved in adipocyte differentiation, demonstrating negatively influence [188, 189]. RBM15B is linked with average daily gain in Italian Large White pigs, while TBPL2 – with fertility [190, 191]. WDHD1 is associated with stillbirth in Large White sows and residual feed intake [79, 181]. According to Gene Ontology terms, BMPR1A is associated with immunity, bone, lung and heart development. BMPR1A is reported to be associated with obesity and important for brown adipocytes, candidate gene affecting the number of thoracic vertebrae in a Large White × Minzhu intercross pigs [138, 192–194]. DOCK3 is linked with fatness and growth in Huainan pigs [195]. LGALS3 is linked with immunity, sensitive to cold exposure, associated with stillbirth, and involved in adipogenesis [181, 196–198]. GLUD1 is an important gene for metabolic process, increased by cold exposure and essential for brown adipocytes [199, 200]. MANF positively regulates thermogenesis, resists obesity, as well as regulates hypothalamic control of food intake and body weight [201–203]. MAPK1IP1L and SOCS4 are likely candidate genes for stillborn [181, 204]. According to Gene Ontology terms, MEF2C is involved in numerous developmental processes, may be a key gene in insulin-induced adipocyte differentiation, involved in fat deposition in pigs, important for foetal developmental, and associated with total number born and number born alive [205–207]. WISP3 is linked with sketetal and muscular development [208]. PDE10A is associated with chest circumference in Yorkshire Pigs, back fat thickness at 100 kg in Landrace pigs, and contributes to the regulation of energy homeostasis [209–211]. PARN was identified as candidate genes associated with age at 100 kg in Large White pigs [212]. TFAM promotes mitochondrial DNA content, which necessary for increased fusion during cold adaptation [213]. Its amount significantly elevated after cold exposure and essential for thermoregulation [214, 215]. Mutation in the TFAM gene effects on fattening and carcass traits in commercial pig populations [216]. TFAM gene expression abundance in particular tissues such as liver and L. dorsi revealed some strong correlations with carcass and meat quality traits including marbling [217]. SNORD22 is associated with trimmed thigh weight in Italian crossbred pigs [218]. U4 and ssc-mir-9-2 were previously determined in pigs [219, 220]. Genes associated with reproductive, meat and fat quality, carcass, and immunity traits in pigs were found in genomic regions affected by putative selection. Along with fatting genes, ones linked with thermogenesis were unexpectedly detected which oppositely should led to fat reduction. However, pigs could not have brown adipocytes but could have beige ones, which are very important for maintaining alternative mechanisms of thermoregulation in pigs that possibly avoid fat reduction [221–224].
CONCLUSION
The dramatic reduction of local pig breeds during last 30 years finally led to 0.56% of the total pig population in the RF, mainly Livni, Altai, and Tsivilsk breeds. There are several reasons for the reduction of local pig breeds: a trend to the reduction the total amount of fat on pork carcass and in meat and the aggressive implementation of the Western commercial breeds. Commercial breeds were bred without taking into account Russia environment, the quality and composition of feed and drinking water. Local pigs bred in the USSR are characterized by unpretentiousness to feed, stress and cold resistance, as well as precocity and high productivity. Livni is one of the Russian local pig breeds. Landrace and the Large White breeds participated in creation of the Livni breed, but obtained breed relationship and admixture results indicated the insignificant participation of these breeds in the formation of the modern allelofund of Livni pigs. The largest amount of common genes was detected between the Livni and Large White breeds. Genes involved in glucose metabolism, namely G6PC2, HKDC1, and HK1 are critical for glucose homeostasis, which could effect on the growth and meat quality traits, as well as on thermogenesis. Other genes were associated with immunity, related to percentage of certain fiber types, growth traits, average daily gain and lean meat percentage, intramuscular fat and backfat thickness, etc. Among 35 common genes of the Livni and Landrace breeds, enrichment with HOXD genes was observed. HOXD genes are involved in various developmental processes, such as single fertilization, skeletal muscle tissue development, adult locomotory behaviour, embryonic skeletal system morphogenesis, lipid metabolism, etc., and are associated with traits such as growth, body weight and composition, fat development, organogenesis and feed intake, etc. Candidate genes associated with various growth, carcass and reproductive traits and essential for thermoregulation were specific to Livni pigs. Livni breed belongs to the meat-and-fat type, but during development pigs could be also meat and fat types. The analysis of genetic architecture confirmed the unique structure of local breed that was bred using commercial Landrace and the Large White breeds. During formation own allelofund, the Livni breed fixed important traits, including flexibility during growing and feeding.
Contribution
I.M. Chernukha conceived and designed the study. I.M. Chernukha, L.V. Fedulova and E.A. Kotenkova designed the methodology. L.V. Fedulova and E.A. Kotenkova analysed and described the results. I.M. Chernukha and E.A. Kotenkova wrote the manuscript. All authors contributed to data interpretation.
CONFLICTS OF INTEREST
The authors declared no conflict of interest regarding the publication of this article.
ACKNOWLEDGEMENTS
The genome-wide SNP genotyping was carried out in L.K. Ernst Federal Research Center for Animal Husbandry, 142132 Podolsk, Russia by A. Abdelmanova, V. Kharzinova and N.A. Zinovieva (Agreement № 95. 2022.223).
FUNDING
The study was carried out within project No. 21-76-20032, supported by the Russian Science Foundation (RSF), https://rscf.ru/en/project/21-76-20032REFERENCES
- Hulsegge I, Calus M, Hoving-Bolink R, Lopes M, Megens H-J, Oldenbroek K. Impact of merging commercial breeding lines on the genetic diversity of Landrace pigs. Genetics Selection Evolution. 2019;51. https://doi.org/10.1186/s12711-019-0502-6
- Ianni A, Bennato F, Martino C, Odoardi M, Sacchetti A, Martino G. Qualitative attributes of commercial pig meat from an Italian native breed: the Nero d’Abruzzo. Foods. 2022;11(9). https://doi.org/10.3390/foods11091297
- Zhao Q, Oyelami FO, Qadri QR, Sun H, Xu Z, Wang Q, et al. Identifying the unique characteristics of the Chinese indigenous pig breeds in the Yangtze River Delta region for precise conservation. BMC Genomics. 2021;22. https://doi.org/10.1186/s12864-021-07476-7
- Zhao Q, López-Cortegano E, Oyelami FO, Zhang Z, Ma P, Wang Q, et al. Conservation priorities analysis of Chinese indigenous pig breeds in the Taihu Lake region. Frontiers in Genetics. 2021;12. https://doi.org/10.3389/fgene.2021.558873
- Panda R, Pawankar KN, Laishram M, Debbarma A. The relevance of pig breeds from North Eastern India towards pork production - A review. International Journal of Advances in Agricultural Science and Technology. 2018;5(7):124–132.
- Touma S, Shimabukuro H, Arakawa A, Oikawa T. Maternal lineage of Okinawa indigenous Agu pig inferred from mitochondrial DNA control region. Asian-Australasian Journal of Animal Sciences. 2019;32(4):501–507. https://doi.org/10.5713/ajas.18.0378
- Muñoz M, Bozzi R, García F, Núñez Y, Geraci C, Crovetti A, et al. Diversity across major and candidate genes in European local pig breeds. PLoS One. 2018;13(11). https://doi.org/10.1371/journal.pone.0207475
- Dadousis C, Muñoz M, Óvilo C, Fabbri MC, Araújo JP, Bovo S, et al. Admixture and breed traceability in European indigenous pig breeds and wild boar using genome-wide SNP data. Scientific Reports. 2022;12. https://doi.org/10.1038/s41598-022-10698-8
- Halimani TE, Mapiye O, Marandure T, Januarie D, Imbayarwo-Chikosi VE, Dzama K. Domestic free-range pig genetic resources in Southern Africa: Progress and prospects. Diversity. 2020;12(2). https://doi.org/10.3390/d12020068
- Osei-Amponsah R, Skinner BM, Adjei DO, Bauer J, Larson G, Affara NA, et al. Origin and phylogenetic status of the local Ashanti Dwarf pig (ADP) of Ghana based on genetic analysis. BMC Genomics. 2017;18. https://doi.org/10.1186/s12864-017-3536-6
- Grossi DA, Jafarikia M, Brito LF, Buzanskas ME, Sargolzaei M, Schenkel FS. Genetic diversity, extent of linkage disequilibrium and persistence of gametic phase in Canadian pigs. BMC Genetics. 2017;18. https://doi.org/10.1186/s12863-017-0473-y
- Willson HE, de Oliveira HR, Schinckel AP, Grossi D, Brito LF. Estimation of genetic parameters for pork quality, novel carcass, primal-cut and growth traits in Duroc pigs. Animals. 2020;10(5). https://doi.org/10.3390/ani10050779
- Cortés O, Martinez AM, Cañon J, Sevane N, Gama LT, Ginja C, et al. Conservation priorities of Iberoamerican pig breeds and their ancestors based on microsatellite information. Heredity. 2016;117:14–24. https://doi.org/10.1038/hdy.2016.21
- Scarpa R, Drucker AG, Anderson S, Ferraes-Ehuan N, Gómez V, Risopatrón CR, et al. Valuing genetic resources in peasant economies: The case of “hairless” creole pigs in Yucatan. Ecological Economics. 2003;45(3):427–443. https://doi.org/10.1016/S0921-8009(03)00095-8
- Koziner AB, Shtakelberg ER. 3Pigs. In: Dmitriev NG, Ernst LK, editors. Animal genetic resources of the USSR. Rome: FAO and UNEP; 1989. pp. 104–153.
- Traspov A, Deng W, Kostyunina O, Ji J, Shatokhin K, Lugovoy S, et al. Population structure and genome characterization of local pig breeds in Russia, Belorussia, Kazakhstan and Ukraine. Genetics Selection Evolution. 2016;48. https://doi.org/10.1186/s12711-016-0196-y
- Development of pig farming in the Russian Federation [Internet]. [cited 2023 Aug 15]. Available from: https://mcx.gov.ru/upload/iblock/22e/22e03f3b762d95fc0b037b9eeede1198.pdf
- Pavlova SV, Kozlova NA, Myshkina MS, Schavlikova TN. Genetic resources of domestic pig-breeding in Russian Federation as of January 1, 2022. Pigbreeding. 2022;(5):9–11. (In Russ.). https://doi.org/10.37925/0039-713X-2022-5-9-11
- Yearbook on breeding work in pig husbandry in establishment of the Russian Federation for 2021 [Internet]. [cited 2023 Aug 17]. Available from: https://vniiplem.com/wp-content/uploads/2023/04/Ezhegodnik-svin.2022.pdf
- Chernukha I, Abdelmanova A, Kotenkova E, Kharzinova V, Zinovieva NA. Assessing genetic diversity and searching for selection signatures by comparison between the indigenous Livni and Duroc breeds in local livestock of the central region of Russia. Diversity. 2022;14(10). https://doi.org/10.3390/d14100859
- Redkin AP. Pig husbandry. Moscow: State Publishing House for Agricultural Literature; 1952. 488 p. (In Russ.).
- Purcell S, Neale B, Todd-Brown K, Thomas L, Ferreira MAR, Bender D, et al. PLINK: A tool set for whole-genome association and population-based linkage analyses. The American Journal of Human Genetics. 2007;81(3):559–575. https://doi.org/10.1086/519795
- Chang CC, Chow CC, Tellier LC, Vattikuti S, Purcell SM, Lee JJ. Second-generation PLINK: Rising to the challenge of larger and richer datasets. GigaScience. 2015;4(1). https://doi.org/10.1186/s13742-015-0047-8
- Keenan K, McGinnity P, Cross TF, Crozier WW, Prodöhl PA. diveRsity: An R package for the estimation of population genetics parameters and their associated errors. Methods in Ecology and Evolution. 2013;4(8):782–788. https://doi.org/10.1111/2041-210X.12067
- Wickham H. ggplot2: Elegant graphics for data analysis. New York: Springer; 2009. 213 p. https://doi.org/10.1007/978-0-387-98141-3
- Weir BS, Cockerham CC. Estimating F-statistics for the analysis of population structure. Evolution. 1984;38(6):1358–1370. https://doi.org/10.2307/2408641
- Huson DH, Bryant D. Application of phylogenetic networks in evolutionary studies. Molecular Biology and Evolution. 2006;23(2):254–267. https://doi.org/10.1093/molbev/msj030
- Alexander DH, Novembre J, Lange K. Fast model-based estimation of ancestry in unrelated individuals. Genome Research. 2009;19:1655–1664. https://doi.org/10.1101/gr.094052.109
- Francis RM. pophelper: An R package and web app to analyse and visualise population structure. Molecular Ecology Resources. 2017;17(1):27–32. https://doi.org/10.1111/1755-0998.12509
- Iso-Touru T, Tapio M, Vilkki J, Kiseleva T, Ammosov I, Ivanova Z, et al. Genetic diversity and genomic signatures of selection among cattle breeds from Siberia, eastern and northern Europe. Animal Genetics. 2016;47(6):647–657. https://doi.org/10.1111/age.12473
- Kijas JW, Lenstra JA, Hayes B, Boitard S, Porto Neto LR, San Cristobal M, et al. Genome-wide analysis of the world’s sheep breeds reveals high levels of historic mixture and strong recent selection. PLoS Biology. 2012;10(2). https://doi.org/10.1371/journal.pbio.1001258
- Zhao F, McParland S, Kearney F, Du L, Berry DP. Detection of selection signatures in dairy and beef cattle using high-density genomic information. Genetics Selection Evolution. 2015;47. https://doi.org/10.1186/s12711-015-0127-3
- Biscarini F, Cozzi P, Gaspa G, Marras G. detectRUNS: Detect runs of homozygosity and runs of heterozygosity in diploid genomes [Internet]. [cited 2023 Aug 17]. Available from: https://cran.r-project.org/web/packages/detectRUNS/index.html
- Ferenčaković M, Sölkner J, Curik I. Estimating autozygosity from high-throughput information: Effects of SNP density and genotyping errors. Genetics Selection Evolution. 2013;45. https://doi.org/10.1186/1297-9686-45-42
- Lencz T, Lambert C, DeRosse P, Burdick KE, Morgan TV, Kane JM, et al. Runs of homo-zygosity reveal highly penetrant recessive loci in schizophrenia. Proceedings of the National Academy of Sciences. 2007;104(50):19942–19947. https://doi.org/10.1073/pnas.0710021104
- Purfield DC, Berry DP, McParland S, Bradley DG. Runs of homozygosity and population history in cattle. BMC Genetic. 2012;13. https://doi.org/10.1186/1471-2156-13-70
- Peripolli E, Stafuzza NB, Munari DP, Lima ALF, Irgang R, Machado MA, et al. Assessment of runs of homozygosity islands and estimates of genomic inbreeding in Gyr (Bos indicus) dairy cattle. BMC Genomics. 2018;19. https://doi.org/10.1186/s12864-017-4365-3
- Grilz-Seger G, Neuditschko M, Ricard A, Velie B, Lindgren G, Mesarič M, et al. Genome-wide homozygosity patterns and evidence for selection in a set of European and Near Eastern horse breeds. Genes. 2019;10(7). https://doi.org/10.3390/genes10070491
- Fariello MI, Boitard S, Naya H, SanCristobal M, Servin B. Detecting signatures of selection through haplotype differentiation among hierarchically structured populations. Genetics. 2013;193(3):929–941. https://doi.org/10.1534/genetics.112.147231
- Scheet P, Stephens M. A fast and flexible statistical model for large-scale population genotype data: Applications to inferring missing genotypes and haplotypic phase. The American Journal of Human Genetics. 2006;78(4):629–644. https://doi.org/10.1086/502802
- Kinsella RJ, Kähäri A, Haider S, Zamora J, Proctor G, Spudich G, et al. Ensembl BioMarts: A hub for data retrieval across taxonomic space. Database. 2011;2011. https://doi.org/10.1093/database/bar030
- Huang DW, Sherman BT, Lempicki RA. Systematic and integrative analysis of large gene lists using DAVID bioinformatics resources. Nature Protocols. 2009;4:44–57. https://doi.org/10.1038/nprot.2008.211
- Richard AJ, Stephens JM. The role of JAK-STAT signaling in adipose tissue function. Biochimica et Biophysica Acta – Molecular Basis of Disease. 2014;1842(3):431–439. https://doi.org/10.1016/j.bbadis.2013.05.030
- Sun WX, Wang HH, Jiang BC, Zhao YY, Xie ZR, Xiong K, et al. Global comparison of gene expression between subcutaneous and intramuscular adipose tissue of mature Erhualian pig. Genetics and Molecular Research. 2013;12(4):5085–5101. https://doi.org/10.4238/2013.October.29.3
- Knights AJ, Liu S, Ma Y, Nudell VS, Perkey E, Sorensen MJ, et al. Acetyl-choline-synthesizing macrophages in subcutaneous fat are regulated by β2 -adrenergic signaling. The EMBO Journal. 2021;40(24). http://dx.doi.org/10.15252/embj.2020106061
- Kim Y, Kang BE, Ryu D, Oh SW, Oh C-M. Comparative transcriptome profiling of young and old brown adipose tissue thermogenesis. International Journal of Molecular Sciences. 2021;22(23). https://doi.org/10.3390/ijms222313143
- Zhao X, Wang C, Wang Y, Lin H, Wang H, Hu H, et al. Comparative gene expression profiling of muscle reveals potential candidate genes affecting drip loss in pork. BMC Genetics. 2019;20. https://doi.org/10.1186/s12863-019-0794-0
- Kollara A, Brown TJ. Expression and function of nuclear receptor co-activator 4: Evidence of a potential role independent of co-activator activity. Cellular and Molecular Life Sciences. 2012;69:3895–3909. https://doi.org/10.1007/s00018-012-1000-y
- Huang M, Zhang H, Wu ZP, Wang XP, Li DS, Liu SJ, et al. Whole-genome resequencing reveals genetic structure and introgression in Pudong White pigs. Animal. 2021;15(10). https://doi.org/10.1016/j.animal.2021.100354
- Somekh J. A methodology for predicting tissue-specific metabolic roles of receptors applied to subcutaneous adipose. Scientific Reports. 2020;10. https://doi.org/10.1038/s41598-020-73214-w
- Samad F, Bai H, Baik N, Haider P, Zhang Y, Rega‐Kaun G, et al. The plasminogen receptor Plg‐RKT regulates adipose function and metabolic homeostasis. Journal of Thrombosis and Haemostasis. 2022;20(3):742–754. https://doi.org/10.1111/jth.15622
- Fan B, Onteru SK, Mote BE, Serenius T, Stalder KJ, Rothschild MF. Large-scale association study for structural soundness and leg locomotion traits in the pig. Genetics Selection Evolution. 2009;41. https://doi.org/10.1186/1297-9686-41-14
- Afonso MS, Verma N, van Solingen C, Cyr Y, Sharma M, Perie L, et al. MicroRNA-33 inhibits adaptive thermogenesis and adipose tissue beiging. Arteriosclerosis, Thrombosis, and Vascular Biology. 2021;41(4):1360–1373. https://doi.org/10.1161/ATVBAHA.120.315798
- Piórkowska K, Żukowski K, Szmatoła T, Ropka-Molik K. Transcript variants of a region on SSC15 rich in QTLs associated with meat quality in pigs. Annals of Animal Science. 2017;17(3):703–715. https://doi.org/10.1515/aoas-2016-0095
- Szántó M, Gupte R, Kraus WL, Pacher P, Bai P. PARPs in lipid metabolism and related diseases. Progress in Lipid Research. 2021;84. https://doi.org/10.1016/j.plipres.2021.101117
- Fu Y, Li C, Tang Q, Tian S, Jin L, Chen J, et al. Genomic analysis reveals selection in Chinese native black pig. Scientific Reports. 2016;6. https://doi.org/10.1038/srep36354
- Zhang W, Li X, Jiang Y, Zhou M, Liu L, Su S, et al. Genetic architecture and selection of Anhui autochthonous pig population revealed by whole genome resequencing. Frontiers in Genetics. 2022;13. https://doi.org/10.3389/fgene.2022.1022261
- Xu J, Ruan Y, Sun J, Shi P, Huang J, Dai L, et al. Association analysis of PRKAA2 and MSMB polymorphisms and growth traits of Xiangsu hybrid pigs. Genes. 2023;14(1). https://doi.org/10.3390/genes14010113
- Xing K, Zhu F, Zhai L, Liu H, Wang Z, Hou Z, et al. The liver transcriptome of two full-sibling Songliao black pigs with extreme differences in backfat thickness. Journal of Animal Science and Biotechnology. 2014;5. https://doi.org/10.1186/2049-1891-5-32
- Dogan AE, Hamid SM, Yildirim AD, Yildirim Z, Sen G, Riera CE, et al. PACT establishes a post-transcriptional brake on mitochondrial biogenesis by promoting the maturation of miR-181c. Journal of Biological Chemistry. 2022;298(7). https://doi.org/10.1016/j.jbc.2022.102050
- Waide EH, Tuggle CK, Serão NVL, Schroyen M, Hess A, Rowland RRR, et al. Genomewide association of piglet responses to infection with one of two porcine reproductive and respiratory syndrome virus isolates. Journal of Animal Science. 2017;95(1):16–38. https://doi.org/10.2527/jas.2016.0874
- Pozhitkov AE, Neme R, Domazet-Lošo T, Leroux BG, Soni S, Tautz D, et al. Tracing the dynamics of gene transcripts after organismal death. Open Biology. 2017;7. https://doi.org/10.1098/rsob.160267
- Guo F, Zhu Y, Han Y, Feng X, Pan Z, He Y, et al. DEPP deficiency contributes to browning of white adipose tissue. International Journal of Molecular Sciences. 2022;23(12). https://doi.org/10.3390/ijms23126563
- Muñoz M, García-Casco JM, Caraballo C, Fernández-Barroso MÁ, Sánchez-Esquiliche F, Gómez F, et al. Identification of candidate genes and regulatory factors underlying intramuscular fat content through longissimus dorsi transcriptome analyses in heavy Iberian pigs. Frontiers in Genetics. 2018;9. https://doi.org/10.3389/fgene.2018.00608
- Liu H, Nguyen YT, Nettleton D, Dekkers JCM, Tuggle CK. Post-weaning blood transcriptomic differences between Yorkshire pigs divergently selected for residual feed intake. BMC Genomics. 2016;17. https://doi.org/10.1186/s12864-016-2395-x
- Do DN, Strathe AB, Ostersen T, Pant SD, Kadarmideen HN. Genome-wide association and pathway analysis of feed efficiency in pigs reveal candidate genes and pathways for residual feed intake. Frontiers in Genetics. 2014;5. https://doi.org/10.3389/fgene.2014.00307
- Chalkias H. Genetic and clinical studies of teat traits in the pig. Doctoral Thesis. Uppsala: Swedish University of Agricultural Science; 2013. 64 p.
- Fan S, Kong C, Chen Y, Zheng X, Zhou R, Zhang X, et al. Copy number variation analysis revealed the evolutionary difference between Chinese Indigenous pigs and Asian wild boars. Genes. 2023;14(2). https://doi.org/10.3390/genes14020472
- Charlton CE, Reeves MA, Brandebourg TD. 140 relaxin regulates porcine adipose tissue development by inhibiting preadipocyte number, stimulating lipolysis, and upregulating mRNA expression of adipokine, fatty acid metabolism and extracellular matrix genes. Journal of Animal Science. 2020;98(Suppl.2):39–40. https://doi.org/10.1093/jas/skz397.090
- Ghebrewold R. Genome-wide association study for the relationship between temperature and feed intake in beef cattle. M.S. Thesis. Lincoln: University of Nebraska; 2018.104 p.
- Zhao YX, Gao GX, Zhou Y, Guo CX, Li B, El-Ashram S, et al. Genome-wide association studies uncover genes associated with litter traits in the pig. Animal. 2022;16(12). https://doi.org/10.1016/j.animal.2022.100672
- Sun H, Wang Z, Zhang Z, Xiao Q, Mawed S, Xu Z, et al. Genomic signatures reveal selection of characteristics within and between Meishan pig populations. Animal Genetics. 2018;49(2):119–126. https://doi.org/10.1111/age.12642
- Landua JD, Moraes R, Carpenter EM, Lewis MT. Hoxd10 is required systemically for secretory activation in lactation and interacts genetically with Hoxd9. Journal of Mammary Gland Biology and Neoplasia. 2020;25:145–62. https://doi.org/10.1007/s10911-020-09454-3
- Clark DL, Boler DD, Kutzler LW, Jones KA, McKeith FK, Killefer J, et al. Muscle gene expression associated with increased marbling in beef cattle. Animal Biotechnology. 2011;22(2):51-63. https://doi.org/10.1080/10495398.2011.552031
- Almeida OAC, Moreira GCM, Rezende FM, Boschiero C, de Oliveira Peixoto J, Ibelli AMG, et al. Identification of selection signatures involved in performance traits in a paternal broiler line. BMC Genomics. 2019;20. https://doi.org/10.1186/s12864-019-5811-1
- Dubey NK, Mishra VK, Dubey R, Deng Y-H, Tsai F-C, Deng W-P. Revisiting the advances in isolation, characterization and secretome of adipose-derived stromal/stem cells. International Journal of Molecular Sciences. 2018;19(8). https://doi.org/10.3390/ijms19082200
- Ahn J, Wu H, Lee K. Integrative analysis revealing human adipose-specific genes and consolidating obesity loci. Scientific Reports. 2019;9. https://doi.org/10.1038/s41598-019-39582-8
- Salavati M, Woolley SA, Cortés Araya Y, Halstead MM, Stenhouse C, Johnsson M, et al. Profiling of open chromatin in developing pig (Sus scrofa) muscle to identify regulatory regions. G3 Genes|Genomes|Genetics. 2022;12(2). https://doi.org/10.1093/g3journal/jkab424
- Messad F, Louveau I, Koffi B, Gilbert H, Gondret F. Investigation of muscle transcriptomes using gradient boosting machine learning identifies molecular predictors of feed efficiency in growing pigs. BMC Genomics. 2019;20. https://doi.org/10.1186/s12864-019-6010-9
- Messad F, Louveau I, Renaudeau D, Gilbert H, Gondret F. Analysis of merged whole blood transcriptomic datasets to identify circulating molecular biomarkers of feed efficiency in growing pigs. BMC Genomics. 2021;22. https://doi.org/10.1186/s12864-021-07843-4
- Kumar V, Sekar M, Sarkar P, Acharya KK, Thirumurugan K. Dynamics of HOX gene expression and regulation in adipocyte development. Gene. 2021;768. https://doi.org/10.1016/j.gene.2020.145308
- Lindholm-Perry AK, Rohrer GA, Kuehn LA, Keele JW, Holl JW, Shackelford SD, et al. Genomic regions associated with kyphosis in swine. BMC Genetics. 2010;11. https://doi.org/10.1186/1471-2156-11-112
- Ferraz ALJ, Ojeda A, López-Béjar M, Fernandes LT, Castelló A, Folch JM, et al. Transcriptome architecture across tissues in the pig. BMC Genomics. 2008;9. https://doi.org/10.1186/1471-2164-9-173
- Esteve-Codina A, Paudel Y, Ferretti L, Raineri E, Megens H-J, Silió L, et al. Dissecting structural and nucleotide genome-wide variation in inbred Iberian pigs. BMC Genomics. 2013;14. https://doi.org/10.1186/1471-2164-14-148
- Wu D-D, Yang C-P, Wang M-S, Dong K-Z, Yan D-W, Hao Z-Q, et al. Convergent genomic signatures of high-altitude adaptation among domestic mammals. National Science Review. 2020;7(6):952–963. https://doi.org/10.1093/nsr/nwz213
- Kyle CJ, Nixon M, Homer NZM, Morgan RA, Andrew R, Stimson RH, et al. ABCC1 modulates negative feedback control of the hypothalamic-pituitary-adrenal axis in vivo in humans. Metabolism. 2022;128. https://doi.org/10.1016/j.metabol.2021.155118
- Nixon M, Mackenzie SD, Taylor AI, Homer NZM, Livingstone DE, Mouras R, et al. ABCC1 confers tissue-specific sensitivity to cortisol versus corticosterone: A rationale for safer glucocorticoid replacement therapy. Science Translational Medicine. 2016;8(352). https://doi.org/10.1126/scitranslmed.aaf9074
- Guo L, Sun H, Zhao Q, Xu Z, Zhang Z, Liu D, et al. Positive selection signatures in Anqing six-end-white pig population based on reduced-representation genome sequencing data. Animal Genetics. 2021;52(2):143–154. https://doi.org/10.1111/age.13034
- Zhang D, He X, Wang W, Liu D. Whole-genome sequencing reveals the genetic relationships and selection signatures of the Min pig. Pakistan Journal of Zoology. 2022;54(3):1187–1198. https://doi.org/10.17582/journal.pjz/20200709040722
- Zhang Z, Wang Z, Yang Y, Zhao J, Chen Q, Liao R, et al. Identification of pleiotropic genes and gene sets underlying growth and immunity traits: A case study on Meishan pigs. Animal. 2016;10(4):550–557. https://doi.org/10.1017/S1751731115002761
- Shi L, Wang L, Liu J, Deng T, Yan H, Zhang L, et al. Estimation of inbreeding and identification of regions under heavy selection based on runs of homozygosity in a Large White pig population. Journal of Animal Science and Biotechnology. 2020;11. https://doi.org/10.1186/s40104-020-00447-0
- Horodyska J, Hamill RM, Varley PF, Reyer H, Wimmers K. Genome-wide association analysis and functional annotation of positional candidate genes for feed conversion efficiency and growth rate in pigs. PLoS ONE. 2017;12(6). https://doi.org/10.1371/journal.pone.0173482
- Mármol-Sánchez E, Quintanilla R, Cardoso TF, Vidal JJ, Amills M. Polymorphisms of the cryptochrome 2 and mitoguardin 2 genes are associated with the variation of lipid-related traits in Duroc pigs. Scientific Reports. 2019;9. https://doi.org/10.1038/s41598-019-45108-z
- Cardoso TF, Quintanilla R, Tibau J, Gil M, Mármol-Sánchez E, González-Rodríguez O, et al. Nutrient supply affects the mRNA expression profile of the porcine skeletal muscle. BMC Genomics. 2017;18. https://doi.org/10.1186/s12864-017-3986-x
- Lee S-H, Kim J-M. Breeding potential for pork belly to the novel economic trait. Journal of Animal Science and Technology. 2023;65(1):1–15. https://doi.org/10.5187/jast.2022.e118
- Baboota RK, Sarma SM, Boparai RK, Kondepudi KK, Mantri S, Bishnoi M. Microarray based gene expression analysis of murine brown and subcutaneous adipose tissue: Significance with human. PLoS ONE. 2015;10(5). https://doi.org/10.1371/journal.pone.0127701
- Lee Y-S, Son S, Lee H-K, Lee RH, Shin D. Elucidating breed-specific variants of native pigs in Korea: Insights into pig breeds' genomic characteristics. Animal Cells and Systems. 2022;26(6):338–347. https://doi.org/10.1080/19768354.2022.2141316
- Ran X, Hu F, Mao N, Ruan Y, Yi F, Niu X, et al. Differences in gene expression and variable splicing events of ovaries between large and small litter size in Chinese Xiang pigs. Porcine Health Management. 2021;7. https://doi.org/10.1186/s40813-021-00226-x
- van Son M, Tremoen NH, Gaustad AH, Myromslien FD, Våge DI, Stenseth E-B, et al. RNA sequencing reveals candidate genes and polymorphisms related to sperm DNA integrity in testis tissue from boars. BMC Veterinary Research. 2017;13. https://doi.org/10.1186/s12917-017-1279-x
- Lee Y-S, Son S, Heo J, Shinm D. Detecting the differential genomic variants using cross-population phenotype-associated variant (XP-PAV) of the Landrace and Yorkshire pigs in Korea. Animal Cells and Systems. 2021;25(6):416–423. https://doi.org/10.1080/19768354.2021.2006310
- Serranito B, Cavalazzi M, Vidal P, Taurisson-Mouret D, Ciani E, Bal M, et al. Local adaptations of Mediterranean sheep and goats through an integrative approach. Scientific Reports. 2021;11. https://doi.org/10.1038/s41598-021-00682-z
- Wang X, Ran X, Niu X, Huang S, Li S, Wang J. Whole-genome sequence analysis reveals selection signatures for important economic traits in Xiang pigs. Scientific Reports. 2022;12. https://doi.org/10.1038/s41598-022-14686-w
- Yonggang L. Differential display reveals a novel pig gene, PRPF3, which is differentially expressed in Large White versus Wujin skeletal muscle tissues. Molecular Biology Reports. 2010;37:2687–2692. https://doi.org/10.1007/s11033-009-9799-5
- Puig-Oliveras A, Ballester M, Corominas J, Revilla M, Estellé J, Fernández AI, et al. A co-association network analysis of the genetic determination of pig conformation, growth and fatness. PLoS ONE. 2014;9(12). https://doi.org/10.1371/journal.pone.0114862
- Jensen KS, Binderup T, Jensen KT, Therkelsen I, Borup R, Nilsson E, et al. FoxO3A promotes metabolic adaptation to hypoxia by antagonizing Myc function. The EMBO Journal. 2011;30(22):4554–4570. https://doi.org/10.1038/emboj.2011.323
- Yu J, Zhou Q-Y, Zhu M-J, Li C-C, Liu B, Fan B, et al. The Porcine FoxO1, FoxO3a and FoxO4 genes: cloning, mapping, expression and association analysis with meat production traits. Asian-Australasian Journal of Animal Sciences. 2007;20(5):627–632. https://doi.org/10.5713/ajas.2007.627
- García-Contreras C, Madsen O, Groenen MAM, López-García A, Vázquez-Gómez M, Astiz S, et al. Impact of genotype, body weight and sex on the prenatal muscle transcriptome of Iberian pigs. PLoS ONE. 2020;15(1). https://doi.org/10.1371/journal.pone.0227861
- Zhang X, Liu Q, Zhang X, Guo K, Zhang X, Zhou Z. FOXO3a regulates lipid accumulation and adipocyte inflammation in adipocytes through autophagy. Inflammation Research. 2021;70:591–603. https://doi.org/10.1007/s00011-021-01463-0
- Zhang B, Qiangba Y, Shang P, Wang Z, Ma J, Wang L, et al. A comprehensive microRNA expression profile related to hypoxia adaptation in the Tibetan pig. PLoS ONE. 2015;10(11). https://doi.org/10.1371/journal.pone.0143260
- Yang Y, Yuan H, Yang T, Li Y, Gao C, Jiao T, et al. The expression regulatory network in the lung tissue of Tibetan pigs provides insight into hypoxia-sensitive pathways in high-altitude hypoxia. Frontiers in Genetics. 2021;12. https://doi.org/10.3389/fgene.2021.691592
- Xing K, Zhao X, Liu Y, Zhang F, Tan Z, Qi X, et al. Identification of differentially expressed microRNAs and their potential target genes in adipose tissue from pigs with highly divergent backfat thickness. Animals. 2020;10(4). https://doi.org/10.3390/ani10040624
- He D, Zou T, Gai X, Ma J, Li M, Huang Z, et al. MicroRNA expression profiles differ between primary myofiber of lean and obese pig breeds. PLoS ONE. 2017;12(7). https://doi.org/10.1371/journal.pone.0181897
- Liu X, Gong J, Wang L, Hou X, Gao H, Yan H, et al. Genome-wide profiling of the microrna transcriptome regulatory network to identify putative candidate genes associated with backfat deposition in pigs. Animals. 2019;9(6). https://doi.org/10.3390/ani9060313
- Gurgul A, Jasielczuk I, Ropka-Molik K, Semik-Gurgul E, Pawlina-Tyszko K, Szmatoła T, et al. A genome-wide detection of selection signatures in conserved and commercial pig breeds maintained in Poland. BMC Genetics. 2018;19. https://doi.org/10.1186/s12863-018-0681-0
- Xu Y, Han Q, Ma C, Wang Y, Zhang P, Li C, et al. Comparative proteomics and phosphoproteomics analysis reveal the possible breed difference in Yorkshire and Duroc boar spermatozoa. Frontiers in Cell and Developmental Biology. 2021;9. https://doi.org/10.3389/fcell.2021.652809
- Mancini C, Gohlke S, Garcia-Carrizo F, Zagoriy V, Stephanowitz H, Schulz TJ. Identification of biomarkers of brown adipose tissue aging highlights the role of dysfunctional energy and nucleotide metabolism pathways. Scientific Reports. 2021;11. https://doi.org/10.1038/s41598-021-99362-1
- Jung SM, Doxsey WG, Le J, Haley JA, Mazuecos L, Luciano AK, et al. In vivo isotope tracing reveals the versatility of glucose as a brown adipose tissue substrate. Cell Reports. 2021;36(4). https://doi.org/10.1016/j.celrep.2021.109459
- Zekri Y, Guyot R, Suñer IG, Canaple L, Stein AG, Petit JV, et al. Brown adipocytes local response to thyroid hormone is required for adaptive thermogenesis in adult male mice. eLife. 2022;11. https://doi.org/10.7554/eLife.81996
- Ponsuksili S, Reyer H, Trakooljul N, Murani E, Wimmers K. Single- and Bayesian multi-marker genome-wide association for haematological parameters in pigs. PLoS ONE. 2016;11(7). https://doi.org/10.1371/journal.pone.0159212
- Paul E, Cronan R, Weston PJ, Boekelheide K, Sedivy JM, Lee S-Y, et al. Disruption of Supv3L1 damages the skin and causes sarcopenia, loss of fat, and death. Mammalian Genome. 2009;20:92–108. https://doi.org/10.1007/s00335-008-9168-z
- Ropka-Molik K, Bereta A, Żukowski K, Tyra M, Piórkowska K, Żak G, et al. Screening for candidate genes related with histological microstructure, meat quality and carcass characteristic in pig based on RNA-seq data. Asian-Australasian Journal of Animal Sciences. 2018;31(10):1565–1574. https://doi.org/10.5713/ajas.17.0714
- Hong J-K, Lee J-B, Ramayo-Caldas Y, Kim S-D, Cho E-S, Kim Y-S, et al. Single-step genome-wide association study for social genetic effects and direct genetic effects on growth in Landrace pigs. Scientific Reports. 2020;10. https://doi.org/10.1038/s41598-020-71647-x
- Zhang Y, O'Keefe RJ, Jonason JH. BMP-TAK1 (MAP3K7) induces adipocyte differentiation through PPARγ signaling. Journal of Cellular Biochemistry. 2017;118(1):204–210. https://doi.org/10.1002/jcb.25626
- Long F, Bhatti MR, Kellenberger A, Sun W, Modica S, Höring M, et al. A low-carbohydrate diet induces hepatic insulin resistance and metabolic associated fatty liver disease in mice. Molecular Metabolism. 2023;69. https://doi.org/10.1016/j.molmet.2023.101675
- Zhou S, Ding R, Meng F, Wang X, Zhuang Z, Quan J, et al. A meta-analysis of genome-wide association studies for average daily gain and lean meat percentage in two Duroc pig populations. BMC Genomics. 2021;22. https://doi.org/10.1186/s12864-020-07288-1
- Xu Z, Sun H, Zhang Z, Zhao Q, Olasege BS, Li Q, et al. Assessment of autozygosity derived from runs of homozygosity in Jinhua pigs disclosed by sequencing data. Frontiers in Genetics. 2019;10. https://doi.org/10.3389/fgene.2019.00274
- Pamenter ME, Hall JE, Tanabe Y, Simonson TS. Cross-species insights into genomic adaptations to hypoxia. Frontiers in Genetics. 2020;11. https://doi.org/10.3389/fgene.2020.00743
- Ramayo-Caldas Y, Ballester M, Fortes MRS, Esteve-Codina A, Castelló A, Noguera JL, et al. From SNP co-association to RNA co-expression: Novel insights into gene networks for intramuscular fatty acid composition in porcine. BMC Genomics. 2014;15. https://doi.org/10.1186/1471-2164-15-232
- Ding Y, Hou Y, Ling Z, Chen Q, Xu T, Liu L, et al. Identification of candidate genes and regulatory competitive endogenous RNA (ceRNA) networks underlying intramuscular fat content in Yorkshire pigs with extreme fat deposition phenotypes. International Journal of Molecular Sciences. 2022;23(20). https://doi.org/10.3390/ijms232012596
- Li W, Yang Y, Liu Y, Liu S, Li X, Wang Y, et al. Integrated analysis of mRNA and miRNA expression profiles in livers of Yimeng black pigs with extreme phenotypes for backfat thickness. Oncotarget. 2017;8:114787–114800. https://doi.org/10.18632/oncotarget.21918
- Diao S, Xu Z, Ye S, Huang S, Teng J, Yuan X, et al. Exploring the genetic features and signatures of selection in South China indigenous pigs. Journal of Integrative Agriculture. 2021;20(5):1359–1371. https://doi.org/10.1016/S2095-3119(20)63260-9
- Falker-Gieske C, Blaj I, Preuß S, Bennewitz J, Thaller G, Tetens J. GWAS for meat and carcass traits using imputed sequence level genotypes in pooled F2-designs in pigs. G3 Genes|Genomes|Genetics. 2019;9(9):2823–2834. https://doi.org/10.1534/g3.119.400452
- Liu X, Wang L, Liang J, Yan H, Zhao K, Li N, et al. Genome-wide association study for certain carcass traits and organ weights in a Large White×Minzhu intercross porcine population. Journal of Integrative Agriculture. 2014;13(12):2721–2730. https://doi.org/10.1016/S2095-3119(14)60787-5
- Sidibeh CO, Pereira MJ, Abalo XM, Boersma GJ, Skrtic S, Lundkvist P, et al. FKBP5 expression in human adipose tissue: Potential role in glucose and lipid metabolism, adipogenesis and type 2 diabetes. Endocrine. 2018;62:116–128. https://doi.org/10.1007/s12020-018-1674-5
- Wang Z, Shang P, Li Q, Wang L, Chamba Y, Zhang B, et al. iTRAQ-based proteomic analysis reveals key proteins affecting muscle growth and lipid deposition in pigs. Scientific Reports. 2017;7. https://doi.org/10.1038/srep46717
- Li L, Xu X, Xiao M, Huang C, Cao J, Zhan S, et al. The profiles and functions of RNA editing sites associated with high-altitude adaptation in goats. International Journal of Molecular Sciences. 2023;24(4). https://doi.org/10.3390/ijms24043115
- Shimada K, Park S, Miyata H, Yu Z, Morohoshi A, Oura S, et al. ARMC12 regulates spatiotemporal mitochondrial dynamics during spermiogenesis and is required for male fertility. Proceedings of the National Academy of Sciences. 2021;118(6). https://doi.org/10.1073/pnas.2018355118
- Liu Q, Yue J, Niu N, Liu X, Yan H, Zhao F, et al. Genome-wide association analysis identified BMPR1A as a novel candidate gene affecting the number of thoracic vertebrae in a Large White × Minzhu intercross pig population. Animals. 2020;10(11). https://doi.org/10.3390/ani10112186
- Ding R, Quan J, Yang M, Wang X, Zheng E, Yang H, et al. Genome-wide association analysis reveals genetic loci and candidate genes for feeding behavior and eating efficiency in Duroc boars. PLoS ONE. 2017;12(8). https://doi.org/10.1371/journal.pone.0183244
- Whittle A, Vidal-Puig A. When BAT is lacking, WAT steps up. Cell Research. 2013;23:868–869. https://doi.org/10.1038/cr.2013.58
- Qian S, Tang Y, Tang Q-Q. Adipose tissue plasticity and the pleiotropic roles of BMP signaling. Journal of Biological Chemistry. 2021;296. https://doi.org/10.1016/j.jbc.2021.100678
- Ou C-Y, Chen T-C, Lee JV, Wang J-C, Stallcup MR. Coregulator cell cycle and apoptosis regulator 1 (CCAR1) positively regulates adipocyte differentiation through the glucocorticoid signaling pathway. Journal of Biological Chemistry. 2014;289:17078–17086. https://doi.org/10.1074/jbc.M114.548081
- Xu Z, Sun H, Zhang Z, Zhang C-Y, Zhao Q, Xiao Q, et al. Selection signature reveals genes associated with susceptibility loci affecting respiratory disease due to pleiotropic and hitchhiking effect in Chinese indigenous pigs. Asian-Australasian Journal of Animal Sciences. 2020;33(2):187–196. https://doi.org/10.5713/ajas.18.0658
- Jankowiak H. Effect of colipase Gene (CLPS) polymorphism on carcass and meat quality in pigs. Folia Biologica. 2005;53(Suppl.1):91–93. https://doi.org/10.3409/173491605775789461
- Kapelański W, Wilkanowska A, Cebulska A, Biegniewska M. The effect of CLPS and RYR1 gene polymorphism on meat quality of Złotnicka spotted pigs. Journal of Central European Agriculture. 2010;11(1):93–98. https://doi.org/10.5513/JCEA01/11.1.817
- Kim H-Y, Caetano-Anolles K, Seo M, Kwon Y, Cho S, Seo K, et al. Prediction of genes related to positive selection using whole-genome resequencing in three commercial pig breeds. Genomics and Informatics. 2015;13(4):137–145. https://doi.org/10.5808/GI.2015.13.4.137
- Liu X, Bai Y, Cui R, He S, Ling Y, Wu C, et al. Integrated analysis of the ceRNA network and M-7474 function in testosterone-mediated fat deposition in pigs. Genes. 2022;13(4). https://doi.org/10.3390/genes13040668
- Dou Y, Qi K, Liu Y, Li C, Song C, Wei Y, et al. Identification and functional prediction of long non-coding RNA in longissimus dorsi muscle of Queshan Black and Large White pigs. Genes. 2023;14(1). https://doi.org/10.3390/genes14010197
- Yudin NS, Larkin DM. Whole genome studies of origin, selection and adaptation of the Russian cattle breeds. Vavilov Journal of Genetics and Breeding. 2019;23(5):559–568. (In Russ.). https://doi.org/10.18699/VJ19.525
- Weldenegodguad M, Popov R, Pokharel K, Ammosov I, Ming Y, Ivanova Z, et al. Whole-genome sequencing of three native cattle breeds originating from the northernmost cattle farming regions. Frontiers in Genetics. 2019;9. https://doi.org/10.3389/fgene.2018.00728
- Chebii VJ, Mpolya EA, Muchadeyi FC, Domelevo Entfellner J-B. Genomics of adaptations in ungulates. Animals. 2021;11(6). https://doi.org/10.3390/ani11061617
- Zhu Y, Li W, Yang B, Zhang Z, Ai H, Ren J, et al. Signatures of selection and interspecies introgression in the genome of Chinese domestic pigs. Genome Biology and Evolution. 2017;9(10):2592–2603. https://doi.org/10.1093/gbe/evx186
- Wang C, Wang H, Zhang Y, Tang Z, Li K, Liu B. Genome-wide analysis reveals artificial selection on coat colour and reproductive traits in Chinese domestic pigs. Molecular Ecology Resources. 2015;15(2):414–424. https://doi.org/10.1111/1755-0998.12311
- Groenen MAM. A decade of pig genome sequencing: a window on pig domestication and evolution. Genetics Selection Evolution. 2016;48. https://doi.org/10.1186/s12711-016-0204-2
- Dong K, Yao N, Pu Y, He X, Zhao Q, Luan Y, et al. Genomic scan reveals loci under altitude adaptation in Tibetan and Dahe pigs. PLoS ONE. 2014;9(10). https://doi.org/10.1371/journal.pone.0110520
- Bovo S, Mazzoni G, Bertolini F, Schiavo G, Galimberti G, Gallo M, et al. Genome-wide association studies for 30 haematological and blood clinical-biochemical traits in Large White pigs reveal genomic regions affecting intermediate phenotypes. Scientific Reports. 2019;9. https://doi.org/10.1038/s41598-019-43297-1
- Buerger F, Müller S, Ney N, Weiner J, Heiker JT, Kallendrusch S, et al. Depletion of Jmjd1c impairs adipogenesis in murine 3T3-L1 cells. Biochimica et Biophysica Acta (BBA) – Molecular Basis of Disease. 2017;1863(7):1709–1717. https://doi.org/10.1016/j.bbadis.2017.05.011
- Rabiee A, Plucińska K, Isidor MS, Brown EL, Tozzi M, Sidoli S, et al. White adipose remodeling during browning in mice involves YBX1 to drive thermogenic commitment. Molecular Metabolism. 2021;44. https://doi.org/10.1016/j.molmet.2020.101137
- Wang Z, Zhao Q, Li X, Yin Z, Chen S, Wu S, et al. MYOD1 inhibits avian adipocyte differentiation via miRNA-206/KLF4 axis. Journal of Animal Science and Biotechnology. 2021;12. https://doi.org/10.1186/s40104-021-00579-x
- Stupka R, Citek J, Sprysl M, Okrouhla M, Brzobohaty L. The impact of MYOG, MYF6 and MYOD1 genes on meat quality traits in crossbred pigs. African Journal of Biotechnology. 2012;11(88):15405–15409. https://doi.org/10.5897/AJB12.1820
- Lee EA, Kim JM, Lim KS, Ryu YC, Jeon WM, Hong KC. Effects of variation in porcine MYOD1 gene on muscle fiber characteristics, lean meat production, and meat quality traits. Meat Science. 2012;92(1):36–43. https://doi.org/10.1016/j.meatsci.2012.03.018
- Guiatti D, Stefanon B, Sgorlon S. Association analysis between single nucleotide polymorphisms in the promoter region of LEP, MYF6, MYOD1, OPN, SCD genes and carcass traits in heavy pigs. Italian Journal of Animal Science. 2013;12(1). https://doi.org/10.4081/ijas.2013.e13
- Cao R, Feng J, Xu Y, Fang Y, Zhao W, Zhang Z, et al. Genomic signatures reveal breeding effects of Lulai pigs. Genes. 2022;13(11). https://doi.org/10.3390/genes13111969
- Braglia S, Davoli R, Zappavigna A, Zambonelli P, Buttazzoni L, Gallo M, et al. SNPs of MYPN and TTN genes are associated to meat and carcass traits in Italian Large White and Italian Duroc pigs. Molecular Biology Reports. 2013;40:6927–6933. https://doi.org/10.1007/s11033-013-2812-z
- Zhai LW, Wang LX, Zhou WL, Wang CD. Association of the MYPN gene polymorphisms with meat quality in commercial pigs. Journal of Animal and Veterinary Advances. 2010;9(4):705–709. https://doi.org/10.3923/javaa.2010.705.709
- Lents CA, Barb CR, Hausman GJ, Nonneman D, Heidorn NL, Cisse RS, et al. Effects of nesfatin-1 on food intake and LH secretion in prepubertal gilts and genomic association of the porcine NUCB2 gene with growth traits. Domestic Animal Endocrinology. 2013;45(2):89–97. https://doi.org/10.1016/j.domaniend.2013.06.002
- Shimizu H, Tanaka M, Osaki A. Transgenic mice overexpressing nesfatin/nucleobindin-2 are susceptible to high-fat diet-induced obesity. Nutrition and Diabetes. 2016;6. https://doi.org/10.1038/nutd.2015.42
- Wang Y, Li Z, Zhang X, Xiang X, Li Y, Mulholland MW, et al. Nesfatin-1 promotes brown adipocyte phenotype. Scientific Reports. 2016;6. https://doi.org/10.1038/srep34747
- Fan S, Liu H, Li L. The REEP family of proteins: Molecular targets and role in pathophysiology. Pharmacological Research. 2022;185. https://doi.org/10.1016/j.phrs.2022.106477
- Favero G, Krajčíková K, Bonomini F, Rodella LF, Tomečková V, Rezzani R. Browning of adipose tissue and sirtuin involvement. In: Szablewski L, editor. Adipose tissue. IntechOpen; 2018. https://doi.org/10.5772/intechopen.74760
- Majeed Y, Halabi N, Madani AY, Engelke R, Bhagwat AM, Abdesselem H, et al. SIRT1 promotes lipid metabolism and mitochondrial biogenesis in adipocytes and coordinates adipogenesis by targeting key enzymatic pathways. Scientific Reports. 2021;11. https://doi.org/10.1038/s41598-021-87759-x
- Ran H, He Q, Han Y, Wang J, Wang H, Yue B, et al. Functional study and epigenetic targets analyses of SIRT1 in intramuscular preadipocytes via ChIP-seq and mRNA-seq. Epigenetics. 2023;18(1). https://doi.org/10.1080/15592294.2022.2135194
- Cao Y, Zhang M, Li Y, Lu J, Zhou W, Li X, et al. O-GlcNAcylation of sirt1 protects against cold stress-induced skeletal muscle damage via amelioration of mitochondrial homeostasis. International Journal of Molecular Sciences. 2022;23(23). https://doi.org/10.3390/ijms232314520
- Rodríguez-Barrueco R, Latorre J, Devis-Jáuregui L, Lluch A, Bonifaci N, Llobet FJ, et al. A microRNA cluster controls fat cell differentiation and adipose tissue expansion by regulating SNCG. Advanced Science. 2022;9(4). https://doi.org/10.1002/advs.202104759
- Du K, Chen G-H, Bai X, Chen L, Hu S-Q, Li Y-H, et al. Dynamics of transcriptome and chromatin accessibility revealed sequential regulation of potential transcription factors during the brown adipose tissue whitening in rabbits. Frontiers in Cell and Developmental Biology. 2022;10. https://doi.org/10.3389/fcell.2022.981661
- Kuehn LA, Rohrer GA, Nonneman DJ, Thallman RM, Leymaster KA. Detection of single nucleotide polymorphisms associated with ultrasonic backfat depth in a segregating Meishan × White Composite population. Journal of Animal Science. 2007;85(5):1111–1119. https://doi.org/10.2527/jas.2006-704
- Sarkar P, Thirumurugan K. New insights into TNFα/PTP1B and PPARγ pathway through RNF213-a link between inflammation, obesity, insulin resistance, and Moyamoya disease. Gene. 2021;771. https://doi.org/10.1016/j.gene.2020.145340
- Pollaci G, Gorla G, Potenza A, Carrozzini T, Canavero I, Bersano A, et al. Novel multifaceted roles for RNF213 protein. International Journal of Molecular Sciences. 2022;23(9). https://doi.org/10.3390/ijms23094492
- Zappaterra M, Gioiosa S, Chillemi G, Zambonelli P, Davoli R. Muscle transcriptome analysis identifies genes involved in ciliogenesis and the molecular cascade associated with intramuscular fat content in Large White heavy pigs. PLoS ONE. 2020;15(5). https://doi.org/10.1371/journal.pone.0233372
- Zeng H, Zhong Z, Xu Z, Teng J, Wei C, Chen Z, et al. Meta-analysis of genome-wide association studies uncovers shared candidate genes across breeds for pig fatness trait. BMC Genomics. 2022;23. https://doi.org/10.1186/s12864-022-09036-z
- Verardo LL, Silva FF, Lopes MS, Madsen O, Bastiaansen JWM, Knol EF, et al. Revealing new candidate genes for reproductive traits in pigs: Combining Bayesian GWAS and functional pathways. Genetics Selection Evolution. 2016;48. https://doi.org/10.1186/s12711-016-0189-x
- Li D, Huang M, Zhuang Z, Ding R, Gu T, Hong L, et al. Genomic analyses revealed the genetic difference and potential selection genes of growth traits in two Duroc lines. Frontiers in Veterinary Science. 2021;8. https://doi.org/10.3389/fvets.2021.725367
- Ma J, Yang J, Zhou L, Zhang Z, Ma H, Xie X, et al. Genome-wide association study of meat quality traits in a White Duroc×Erhualian F2 intercross and Chinese Sutai pigs. PLoS ONE. 2013;8(5). https://doi.org/10.1371/journal.pone.0064047
- Wang BB, Hou LM, Zhou WD, Liu H, Tao W, Wu WJ, et al. Genome-wide association study reveals a quantitative trait locus and two candidate genes on Sus scrofa chromosome 5 affecting intramuscular fat content in Suhuai pigs. Animal. 2021;15(9). https://doi.org/10.1016/j.animal.2021.100341
- Li M, Tian S, Jin L, Zhou G, Li Y, Zhang Y, et al. Genomic analyses identify distinct patterns of selection in domesticated pigs and Tibetan wild boars. Nature Genetics. 2013;45:1431–1438. https://doi.org/10.1038/ng.2811
- Im D-S. Discovery of new G protein-coupled receptors for lipid mediators. Journal of Lipid Research. 2004;45(3):410–418. https://doi.org/10.1194/jlr.R300006-JLR200
- Hlongwane NL, Hadebe K, Soma P, Dzomba EF, Muchadeyi FC. Genome wide assessment of genetic variation and population distinctiveness of the pig family in South Africa. Frontiers in Genetics. 2020;11. https://doi.org/10.3389/fgene.2020.00344
- Hamza MS, Pott S, Vega VB, Thomsen JS, Kandhadayar GS, Ng PWP, et al. De-novo identification of PPARγ/RXR binding sites and direct targets during adipogenesis. PLoS ONE. 2009;4(3). https://doi.org/10.1371/journal.pone.0004907
- Honda T, Ishii A, Inui M. Regulation of adipocyte differentiation of 3T3-L1 cells by PDZRN3. American Journal of Physiology-Cell Physiology. 2013;304(11):C1091–S1097. https://doi.org/10.1152/ajpcell.00343.2012
- Fontanesi L, Schiavo G, Galimberti G, Calò DG, Russo V. A genomewide association study for average daily gain in Italian Large White pigs. Journal of Animal Science. 2014;92(4):1385–1394. https://doi.org/10.2527/jas.2013-7059
- Oqani RK, So S, Lee Y, Ko JJ, Kang E. Artificial oocyte: Development and potential application. Cells. 2022;11(7). https://doi.org/10.3390/cells11071135
- Böttcher Y, Unbehauen H, Klöting N, Ruschke K, Körner A, Schleinitz D, et al. Adipose tissue expression and genetic variants of the bone morphogenetic protein receptor 1A gene (BMPR1A) are associated with human obesity. Diabetes. 2009;58(9):2119–2128. https://doi.org/10.2337/db08-1458
- Keipert S, Kutschke M, Ost M, Schwarzmayr T, van Schothorst EM, Lamp D, et al. Long-term cold adaptation does not require FGF21 or UCP1. Cell Metabolism. 2017;26(2):437–446. https://doi.org/10.1016/j.cmet.2017.07.016
- Schulz TJ, Huang P, Huang TL, Xue R, McDougall LE, Townsend KL, et al. Brown-fat paucity due to impaired BMP signalling induces compensatory browning of white fat. Nature. 2013;495:379–383. https://doi.org/10.1038/nature11943
- Qiao R, Zhang M, Zhang B, Li X, Han X, Wang K, et al. Population genetic structure analysis and identification of backfat thickness loci of Chinese synthetic Yunan pigs. Frontiers in Genetics. 2022;13. https://doi.org/10.3389/fgene.2022.1039838
- Burl RB, Rondini EA, Wei H, Pique-Regi R, Granneman JG. Deconstructing cold-induced brown adipocyte neogenesis in mice. eLife. 2022;11. https://doi.org/10.7554/eLife.80167
- Poklukar K, Čandek-Potokar M, Vrecl M, Batorek-Lukač N, Fazarinc G, Kress K, et al. Adipose tissue gene expression of entire male, immunocastrated and surgically castrated pigs. International Journal of Molecular Sciences. 2021;22(4). https://doi.org/10.3390/ijms22041768
- Blasetti Fantauzzi C, Iacobini C, Menini S, Vitale M, Sorice GP, Mezza T, et al. Galectin-3 gene deletion results in defective adipose tissue maturation and impaired insulin sensitivity and glucose homeostasis. Scientific Reports. 2020;10. https://doi.org/10.1038/s41598-020-76952-z
- Wang GX, Meyer JG, Cai W, Softic S, Li ME, Verdin E, et al. Regulation of UCP1 and mitochondrial metabolism in brown adipose tissue by reversible succinylation. Molecular Cell. 2019;74(4):844–857. https://doi.org/10.1016/j.molcel.2019.03.021
- Okamatsu-Ogura Y, Kuroda M, Tsutsumi R, Tsubota A, Saito M, Kimura K, et al. UCP1-dependent and UCP1-independent metabolic changes induced by acute cold exposure in brown adipose tissue of mice. Metabolism. 2020;113. https://doi.org/10.1016/j.metabol.2020.154396
- Wen X, Zhang B, Wu B, Xiao H, Li Z, Li R, et al. Signaling pathways in obesity: Mechanisms and therapeutic interventions. Signal Transduction and Targeted Therapy. 2022;7. https://doi.org/10.1038/s41392-022-01149-x
- Tang Q, Liu Q, Li J, Yan J, Jing X, Zhang J, et al. MANF in POMC neurons promotes brown adipose tissue thermogenesis and protects against diet-induced obesity. Diabetes. 2022;71(11):2344–2359. https://doi.org/10.2337/db21-1128
- Yang S, Yang H, Chang R, Yin P, Yang Y, Yang W, et al. MANF regulates hypothalamic control of food intake and body weight. Nature Communications. 2017;8. https://doi.org/10.1038/s41467-017-00750-x
- Sell-Kubiak E, Dobrzanski J, Derks MFL, Lopes MS, Szwaczkowski T. Meta-analysis of SNPs determining litter traits in pigs. Genes. 2022;13(10). https://doi.org/10.3390/genes13101730
- Li R, Meng S, Ji M, Rong X, You Z, Cai C, et al. HMG20A inhibit adipogenesis by transcriptional and epigenetic regulation of MEF2C expression. International Journal of Molecular Sciences. 2022;23(18). https://doi.org/10.3390/ijms231810559
- Ren H, Xiao W, Qin X, Cai G, Chen H, Hua Z, et al. Myostatin regulates fatty acid desaturation and fat deposition through MEF2C/miR222/SCD5 cascade in pigs. Communications Biology. 2020;3. https://doi.org/10.1038/s42003-020-01348-8
- Onteru SK, Fan B, Du Z-Q, Garrick DJ, Stalder KJ, Rothschild MF. A whole-genome association study for pig reproductive traits. Animal Genetics. 2012;43(1):18–26. https://doi.org/10.1111/j.1365-2052.2011.02213.x
- Gautier M, Moazami-Goudarzi K, Levéziel H, Parinello H, Grohs C, Rialle S, et al. Deciphering the wisent demographic and adaptive histories from individual whole-genome sequences. Molecular Biology and Evolution. 2016;33(11):2801–2814. https://doi.org/10.1093/molbev/msw144
- Liu H, Song H, Jiang Y, Jiang Y, Zhang F, Liu Y, et al. A Single-Step Genome wide association study on body size traits using imputation-based whole-genome sequence data in Yorkshire pigs. Frontiers in Genetics. 2021;12. https://doi.org/10.3389/fgene.2021.629049
- Chen D, Wu P, Yang Q, Wang K, Zhou J, Yang X, et al. Genome-wide association study for backfat thickness at 100 kg and loin muscle thickness in domestic pigs based on genotyping by sequencing. Physiological Genomics. 2019;51(7):261–266. https://doi.org/10.1152/physiolgenomics.00008.2019
- Martínez-Montes ÁM, Fernández A, Muñoz M, Noguera JL, Folch JM, Fernández AI. Using genome wide association studies to identify common QTL regions in three different genetic backgrounds based on Iberian pig breed. PLoS ONE. 2018;13(3). https://doi.org/10.1371/journal.pone.0190184
- Yang W, Wu J, Yu J, Zheng X, Kang H, Wang Z, et al. A genome-wide association study reveals additive and dominance effects on growth and fatness traits in large white pigs. Animal Genetics. 2021;52(5):749–753. https://doi.org/10.1111/age.13131
- Bal NC, Maurya SK, Singh S, Wehrens XHT, Periasamy M. Increased reliance on muscle-based thermogenesis upon acute minimization of brown adipose tissue function. Journal of Biological Chemistry. 2016;291(33):17247–17257. https://doi.org/10.1074/jbc.M116.728188
- Lu Y, Fujioka H, Joshi D, Li Q, Sangwung P, Hsieh P, et al. Mitophagy is required for brown adipose tissue mitochondrial homeostasis during cold challenge. Scientific Reports. 2018;8. https://doi.org/10.1038/s41598-018-26394-5
- Tabuchi C, Sul HS. Signaling pathways regulating thermogenesis. Frontiers in Endocrinology. 2021;12. https://doi.org/10.3389/fendo.2021.595020
- Kunej T, Wu X-L, Michal JJ, Berlic TM, Jiang Z, Dovc P. The porcine mitochondrial transcription factor a gene: Molecular characterization, radiation hybrid mapping and genetic diversity among 12 pig breeds. American Journal of Animal and Veterinary Sciences. 2009;4(4):129–135.
- Song Q, Zhang W, Wu F, Xu N, Zhang J, Xu M, et al. Cloning and expression levels of TFAM and TFB2M gene and their correlation with meat and carcass quality traits in Jiaxing Black Pig. Annals of Animal Science. 2019;19(2):327–341. https://doi.org/10.2478/aoas-2018-0056
- Palombo V, D’Andrea M, Licastro D, Dal Monego S, Sgorlon S, Sandri M, et al. Single-step genome wide association study identifies QTL signals for untrimmed and trimmed thigh weight in Italian crossbred pigs for dry-cured ham production. Animals. 2021;11(6). https://doi.org/10.3390/ani11061612
- Li M, Xia Y, Gu Y, Zhang K, Lang Q, Chen L, et al. MicroRNAome of porcine pre- and postnatal development. PLoS ONE. 2010;5(7). https://doi.org/10.1371/journal.pone.0011541
- Anthon C, Tafer H, Havgaard JH, Thomsen B, Hedegaard J, Seemann SE, et al. Structured RNAs and synteny regions in the pig genome. BMC Genomics. 2014;15. https://doi.org/10.1186/1471-2164-15-459
- Fyda TJ, Spencer C, Jastroch M, Gaudry MJ. Disruption of thermogenic UCP1 predated the divergence of pigs and peccaries. Journal of Experimental Biology. 2020;223(15). https://doi.org/10.1242/jeb.223974
- Jastroch M, Andersson L. When pigs fly, UCP1 makes heat. Molecular Metabolism. 2015;4(5):359–362. https://doi.org/10.1016/j.molmet.2015.02.005
- Zhao J, Tao C, Chen C, Wang Y, Liu T. Formation of thermogenic adipocytes: What we have learned from pigs. Fundamental Research. 2021;1(4):495–502. https://doi.org/10.1016/j.fmre.2021.05.004
- Lin J, Cao C, Tao C, Ye R, Dong M, Zheng Q, et al. Cold adaptation in pigs depends on UCP3 in beige adipocytes. Journal of Molecular Cell Biology. 2017;9(5):364–375. https://doi.org/10.1093/jmcb/mjx018