Abstract
There are two main types of radiation: ionizing and non-ionizing. Radiations are widely distributed in the earth’s crust with small amounts found in water, soil, and rocks. Humans can also produce them through military, scientific, and industrial activities. Ionizing and nonionizing radiations have a wide application in the food industry and medicine. γ-rays, X-rays, and electron beams are the main sources of radiation used in the food industry for food processing. This review discusses advantages and disadvantages of ionizing radiation on microorganisms and its potential applications in the food industry. We also looked at its advantages and disadvantages.Studies have revealed that ionizing radiation is used in the food industry to inactivate microorganisms in food products to improve hygiene, safety, and extend shelf life. Microorganisms such as bacteria and fungi are susceptible to high doses of irradiation. However, some bacterial and fungal species have developed an exceptional ability to withstand the deleterious effect of radiation. These organisms have developed effective mechanisms to repair DNA damage resulting from radiation exposure.
Currently, radiation has become a promising technology for the food industry, since fruits, tubers, and bulbs can be irradiated to delay ripening or prevent sprouting to extend their shelf life.
Keywords
Ionizing radiation, activation, inactivation, food products, application, shelf life, microorganismsINTRODUCTION
Ionizing radiation is a form of electromagnetic waves or particles which possess a significant amount of energy capable of ionizing an atom or a molecule. Too much exposure to ionizing radiation is harmful to living organisms. Some microorganisms have developed the capacity to survive in radioactive environments [1]. Living organisms are constantly faced with the challenge of surviving under extreme environmental conditions. Diverse groups of microorganisms such as bacteria, archaea, and eukaryotes can survive in harsh environments such as intense ultraviolet (UV) radiation, extreme temperatures, and desiccation. These conditions provide a lot of selection pressure for extre- mophiles [2].
Ionizing particles such as neutrons or a-particles can disrupt the DNA structure through a direct interaction with DNA, which can damage the sugar backbone and base pairs [3]. Radiation can induce genomic instability in living organisms by introducing either DNA single-strand breaks or double-strand breaks. When unrepaired, these errors may lead to cell death [4-6]. Reactive oxygen species produced by ionizing radiation in microorganisms can damage cellular macromolecules such as nucleic acids, proteins, lipids, and carbohydrates [7]. Post-translational addition of carbonyl groups to amino acid side chains increases after exposure to ionizing radiation. This results in a loss of protein function due to changes in protein folding [8, 9]. In other to overcome the deleterious effect of ionizing radiation, living organisms activate enzymatic and non-enzymatic antioxidant defense systems, DNA repair mechanisms, induction of protein folding, and degradation processes [10].
Irradiation food processing is a non-thermal method of administering specific doses of radiation to destroy microorganisms in food, thus enhancing its hygiene and safety and prolonging its shelf life [11]. The use of irradiation in food processing is highly recognized and well regulated. It can be applied to foods ranging from dried products like seasonings and spices to moisture-containing food such as meat. The presence of pathogens in fresh foods can cause foodborne illnesses. Irradiation technologies can decontaminate vegetables and fresh fruits without subjecting them to heat treatments [11]. Thermal methods such as sterilization and pasteurization have been effectively used to reduce fungi and mycotoxins in food products. However, processing at high temperatures can have a detrimental effect on the nutritional and sensory properties of food products. Therefore, we need to explore other technologies such as radiation [12]. In this review, we aimed to explore the effects of radiation on microorganisms and its applications in the food industry.
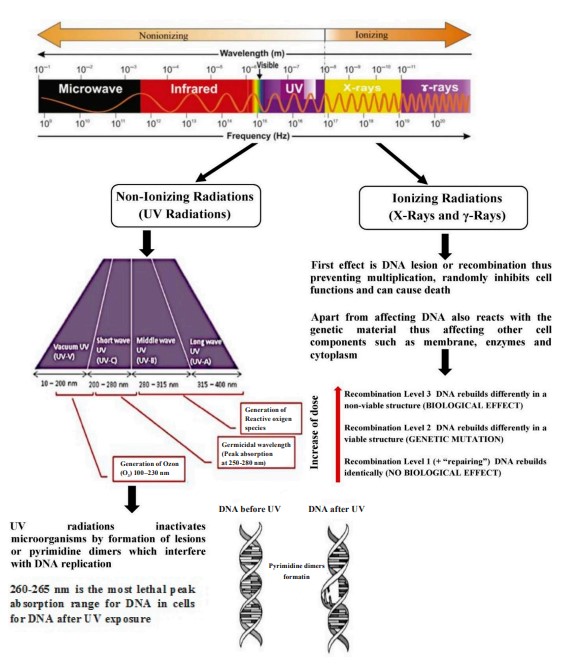
RESULTS AND DISCUSSION
Radiation sources. Electromagnetic radiation is energy that travels at a constant speed through a sinusoidal path in different magnetic and electric fields. Its electromagnetic spectrum has different energies stretching from long-wavelength radio waves to shortwavelength y-rays. In between these two limits, there are other forms of radiation such as microwave, infrared, visible light, ultraviolet, and X-ray [13]. There are two kinds of radiation, namely ionizing and non-ionizing. Non-ionizing radiation is a type of energy which can be generated by an instrument or machine in the form of electromagnetic waves with a specific wavelength [11]. Ionizing radiation, on the other hand, is a type of energy with a very short wavelength but high intensity capable of removing electrons from atoms. Its forms include X-rays, electron beams, and y-rays [11, 13].
Radiations can emerge from both natural and artificial sources. Those from natural sources are due to the presence of several radiating elements found in the earth’s crust, while those from artificial sources emerge from human scientific, military, and industrial activities [14]. The earliest radionuclides comprise uranium 238, thorium 232, potassium 40, radium 226, etc. They are mostly found in soils, rocks, and in many building materials. A good amount of environmental contamination with radioactive substances owes its source to nuclear power plant accidents, nuclear waste disposal, nuclear power testing, and mismanagement of radioactive materials [15].
Food processing can only use y-radiation, X-rays, and accelerated electrons since other particles can induce radioactivity. Electron beam radiation is produced by an electron gun from which high-energy electron beams are emitted. A low penetration capacity (limited to approximately 2.5 cm) is a major drawback of applying electron beams to food. Therefore, this type of radiation cannot be used for a wide variety of foods. X-rays are high-energy photons that are also generated from machine sources [16]. y-radiations have a higher photon energy with a shorter wavelength. They are primarily produced from the decay of Cobalt-60 (60Co) or Cesium 137 (137Cs). 60Co is obtained from loading small pellets of cobalt into sealed stainless steel or zirconium alloy tubes. 60Co radionuclide is produced by the irradiation of 59Co in a nuclear reactor by fast neutrons [17].
The effect of radiation on bacteria. Radiation, such as UV, causes indirect damage to proteins, lipids, and DNA, resulting in the formation of reactive oxygen species in bacteria. Radiation damages the bacterial DNA by stimulating single-strand breaks and causing pyrimidine dimers to form. Bacteria have many repair mechanisms in response to the damage caused by radiation. Some of them are post-replication recombination, nucleotide excision, and error-prone repairs [18, 19].
Bacteria belonging to the Deinococcaceae family are able to survive the deleterious effect of ionizing radiation. Among these radiation-resistant organisms is Deinococcus radiodurans [2, 20]. After exposing D. radiodurans to approximately 7 kGy of ionizing radiation, its genome was broken into fragments, but it did not have any effect on its survival [20].
There are no studies to justify a higher content of proteins involved in the double-strand breaks repair (such as RecA and PolA) in D. radiodurans than in other bacteria species such as Escherichia coli [21, 22]. Research shows that the ability of D. radiodurans to withstand radiation and desiccation is inherent in its Mn-dependent mechanisms which protect proteins from oxidative modifications that introduce carbonyl groups. The effect of y-radiation on bacteria leads to a rise in protein carbonylation, which inhibits the catalytic activity of proteins and promotes cell death [10]. D. radiodurans bacteria possess about 300 times more Mn (II) ions than ionizing-radiation-sensitive bacteria such as Thermus thermophiles and E. coli. Due to high levels of intracellular Mn (II) ions, which protect proteins, D. radiodurans can resist the deleterious effect of 10 kGy ionizing radiation [23].
At the molecular and biological level, radiation targets the bacterial DNA, proteins, and cell membranes. The degree of damage depends on the genetic composition of bacteria. Each bacteria strain has several parameters which affect the yield of lesions per unit dose of radiation and the ability to cause cell death. Differences in sensitivity to radiation in different types of bacteria depend on the effectiveness of their cellular DNA repair mechanisms [24].
The effect of radiation on yeast. Yeasts are eukaryotic microorganisms which belong to the fungi kingdom. Saccharomyces cerevisiae is a type of yeast mostly used in the food industry for baking and brewing. Other species include Candida, Saccharomyces, Debaryomyces, Hanseniaspora, Dekkera, Kluyveromyces, Rhodotorula, Meyerozyma, Pichia, Zygosaccharomyces, and Torulaspora [25].
A study conducted by Takeshita et al. exposed yeast cells to pulsed light and UV irradiation and showed differences in the proteins eluted from the yeast cells [19]. The elution of proteins is a possible indication of cell damage induced by irradiation. Pulse light irradiation is known to cause structural changes in yeast cells, enlarging vacuoles and distorting cell shape and cell membrane, as observed under transmission electron microscopy. These structural changes suggest that irradiation can cause damage to yeast cell membranes [19]. Ionizing radiation causes cellular damage to DNA, such as base damage, single-strand breaks, strand crosslinks, and double-strand breaks [26].
Budding yeasts exhibit resistance to ionizing radiation in the synthesis (S) and mitosis (M) phases than nonbudding yeasts in the Gap 1 phase. Growing yeast cells in the synthesis/Gap 2 exhibit greater X-ray-induced instability than the stationary phase Gap 1/Gap 0 (G1/G0) yeast cells. It has been shown that yeast cells in the Gap 1 (G1) phase are more sensitive to mutations caused by radiation, less sensitive in the early synthesis, and least sensitive in the late synthesis/ Gap 2 (S/G2) [27]. Yeast cells exhibit varied sensitivity to radiation at different growth stages. They are more resistant to radiation in the stationary phase (this resistance is different from what is observed in the cell cycle). However, cells are more sensitive to radiation in the exponential growth phase when the majority of cells are in the synthesis/Gap 2 phase. Studies have shown that budding yeasts are more resistant to the killing effect of radiation than nonbudding yeasts [28-30]. Also, yeasts show much greater resistance to environmental stressors than prokaryotes because of their ecological versatility [31]. Yeast cell membrane and DNA are susceptible to radiation damage and inactivation. There is a lack of information to support the effect of radiation on yeasts. Therefore, more studies are needed to show what damage is caused by their exposure to radiation.The effect and response of fungi to radiation. D. radiodurans bacterium is known to withstand higher levels of ionizing radiation. However, studies have also identified some fungal species with a greater potential to withstand the effects of radiation compared to this bacterium. After the explosion at the Chernobyl nuclear power plant in 1986, and several years down the line, over 2000 fungal species were isolated in that area [31]. Some of the species were remarkably radioresistant, including melanized fungal species (Cladosporium spp.), Cryomyces antarcticus, Aureobasidium, and yeast [31]. Melanized fungi were predominant compared to the other fungal species [32, 33].
A study by Dadachova et al. showed that melanized fungal species, such as Cryptococcus neoformans and Wangiella dermatitidis, experienced a higher cell growth compared to their non-melanized counterparts [34]. The authors found that ionizing radiation interfered with the electron transfer properties in melanin by analyzing the NADH-ferricyanide redox reaction. This suggests that melanized fungi can use radiation (y-radiation) as an energy source to convert electromagnetic energy into chemical energy that the fungi can utilize [10]. Melanin protects fungal cells from various environmental stresses, including oxidative damage, heavy metals, and UV irradiation [35]. It plays an important role in radiation resistance by dispersing electrons and photons and serving as a radioprotective barrier against radiation and scavenging radiation-induced reactive oxygen species [36].
Transcriptome analysis was conducted by Jung et al. to explain the mechanism of radiation resistance in C. neoformans [37]. They found a significant variation in the C. neoformans genes. Out of 6962 identified genes, 37% showed different expression patterns after y-radiation exposure. The genes involved in posttranslational modification, DNA replication and repair, as well as protein turnover and chaperone function, were extremely upregulated during the early recovery time. However, the genes that participated in translation, transport, and amino acid metabolism were downregulated in the late recovery time. The transcriptome analysis of C. neoformans was similar to that of radiation-sensitive fungi such as Schizosaccharomyces pombe and S. cerevisiae [38, 39].
Fungi have developed several effective mechanisms to withstand the deleterious effect of radiation compared to other organisms. The genes involved in DNA damage repair and oxidative stress responses are upregulated during radiation exposure. Fungi produce protective molecules such as melanin, carotenoids, trehalose 1,3-glucan, and others. These molecules contribute to the fungi’s exceptional ability to protect themselves against radiation and other harmful environmental stresses, such as dryness and salinity [31]. There is limited information in literature on the effects of radiation on fungi. Therefore, more research is needed to elucidate what kind of mechanisms are employed by fungi to overcome the deleterious effects of ionizing radiation.
Mechanisms of microbial inactivation. Ionizing and non-ionizing radiations have different effects on microorganisms because of the different energies they possess. Ionizing radiation produces free radicals in microorganisms and causes damage to genetic materials and other cellular components, contributing to cell death. However, microbial inactivation or destruction by non-ionizing radiation is facilitated by the thermal decomposition of proteins, lipids, and DNA, leading to cell rupture or disintegration. Spores produced by bacteria and fungi are resistant to irradiation because they contain a small amount of DNA and are very stable. Microorganisms respond differently to radiation due to differences in their physical and chemical structures and in their spore-producing capacity [13].
Ionizing radiation can destroy microbes and mycotoxins employing two major mechanisms, namely the direct interaction with the organism and the indirect action by water radiolysis. The direct interaction of radiation causes damage to genetic materials. In fungi, for instance, this can lead to the death of living cells, preventing the formation and release of mycotoxins. In the indirect method, radiation reacts with water molecules (radiolysis) to produce positively-charged water radicals (H2O+) and negative free solvated electrons (e-). These interactions result in the formation of several reactive species such as H+, OH+, H3O+, H2O2, and OH-. Subsequently, these free radicals attack cytoplasmic components and destroy organic molecules. The hydroxyl free radical removes hydrogen atoms from the bases within the DNA strands, causing death to organisms which cannot recover from this DNA damage [13]. Many studies have identified damage of deoxyribonucleic or ribonucleic acids coupled with the disintegration of cytoplasmic membranes as major mechanisms for microbial inactivation by ionizing radiation. The induction of single-strand breaks is not very destructive, but double- or multiplestrand breaks can render the microbe inactive [40]. Figure 2 shows how radiation can damage the DNA in microorganisms.
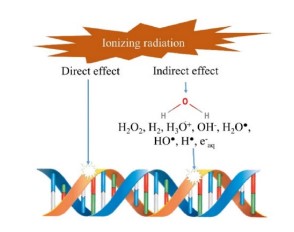
Justification of ionizing radiation in food processing. Food irradiation is a non-thermal method of exposing food to specific doses of non-ionizing or ionizing radiations to destroy microorganisms (bacteria, fungi, viruses, etc.) in food or agricultural products to improve their hygiene and safety, as well as prolong their shelf life [42, 43]. The use of radiation technology in food processing has proven to be effective and it is widely used for various kinds of foodstuffs from dried to moisturized food products [44]. The rise in different kinds of food-borne diseases caused by consuming fresh foods contaminated with pathogens has made food irradiation an alternative approach to decontaminating fresh vegetables and fruits without heat treatments using sterilization or pasteurization [45].
Mycotoxin contamination of food products is raising several global concerns because it affects human health, causes economic loss to farmers, and leads to food wastage [46, 47]. Mycotoxins are produced in food contaminated with fungi. This can result in mycotoxin poisoning and various health consequences, such as gynecomastia, esophageal cancer, human nephropathies, hepatocellular carcinoma, liver cancers, hepatitis B, reduced levels of immunoglobulins, as well as increased morbidity and mortality [48-50].Thermal food preservation methods used to eliminate enzymes, mycotoxins, and pathogenic microorganisms consume a lot of energy, thereby increasing the cost of preservation. Thermal treatment methods also cause loss of certain nutrients (vitamins, proteins, etc.) and sensory properties, affecting the overall quality of the food product [51-53]. To overcome this negative impact, the food industry is exploring alternative processing techniques, such as cold plasma, high pressure processing, and irradiation technologies [11].
Using electromagnetic radiation as an energy source is a better option to ensure microbial safety, improve sensory properties, and retain nutrients [54]. It does not leave residues and toxins in the product. Nor does it affect the odor, pH, flavor, or taste [55]. Parasites, such as Toxoplasma gondii, Cysticercus spp., Taenia solium, Trichinella spiralis, parasitic protozoa, and helminths in meat and fish, can be inactivated through 0.3 to 1 kGy irradiation to guarantee the product’s safety for consumers [56].
Applications of ionizing radiation in the food industry. Food irradiation is a method used in food processing and preservation. This involves administering certain amounts of ionizing radiation to food in a radiation-protected chamber [57]. Radiation can be applied to food products to either pasteurize or sterilize them to reduce or eliminate pathogens and other microorganisms, except certain viruses, based on the radiation dose [16]. Microorganisms, such as E. coli, Campylobacter, Salmonella, and others, can be removed from food by irradiation to improve the product’s shelf-life and guarantee safety. Sterile foods are very important for consumers, especially patients with a compromised or suppressed immune system who need to consume bacteria-free foods [16]. Practical doses of radiation given to food are classified into three main categories, as shown in Fig. 3 [56].
The focus of using this technology in the food industry is to maintain stability in stored products, eliminate insects or parasites, inhibit the activity of enzymes, and reduce food-borne contaminants such as bacteria, viruses, fungi, and mycotoxins [13]. The allowable dose of radiation delivered to food should not exceed 10 kGy, since this dose does not compromise the product’s safety or affect its structure and functional properties [40]. Some applications of radiation technology in the food industry:
– irradiation of spices and condiments;
– control of sprouting in tubers and bulbs;
– insect disinfection in cereals;
– fruits and vegetables;
– fish and meat products; and
– application of irradiation in dairy products.
Irradiation of spices and condiments. Spices and condiments are naturally contaminated with numerous microorganisms or due to poor harvest and storage conditions [58]. Spices can be contaminated with pathogenic microorganisms such as Clostridium perfringens, Bacillus cereus, Salmonella, E. coli, and toxigenic molds. Several studies have confirmed that up to 10 kGy of irradiation can reduce the microbial load without any adverse chemical or organoleptic changes in spices [56, 59].
The use of spices to season processed foods without sterilization may increase food spoilage and even cause food-borne diseases. In the past, fumigants (methyl bromide and ethylene oxide) were used for insect, bacteria, and mold elimination because moist heat treatment was not suitable for dried products. However, these chemicals are highly toxic and raise safety and environmental concerns. Spice irradiation has been approved by many countries in recent times and the food industry is moving in this direction. Doses of 5-10 kGy are enough to eliminate mold spores, bacteria, and insects without any effects on the sensory or chemical properties of spices [60].
Control of sprouting in tubers and bulbs. Sprouting affects the shelf life of potatoes, garlic, onions, ginger shallots, and yam. These agricultural products have to be stored for several months to ensure adequate supplies to consumers, but due to sprouting, large losses are observed after harvesting [56]. Irradiation has proven to be effective for shelf-life extension [45, 61]. Low radiation doses of 0.02-0.2 and 0.25-1.0 kGy are used to prevent the sprouting of bulbs and tubers, respectively [56].
Sprouting and rotting of tubers and bulbs occur when they are stored under low temperatures (4-20°C) and high humidity (> 85%) [62]. Sprouting of tuber crops can be reduced by applying lower radiation doses (0.15 kGy) during the dormancy period. Although the dose needed for sprout prevention does not affect the physicochemical properties of the produce, in certain cases brownish discoloration can occur in the inner buds [17]. In a study by Tripathi et al., several onion varieties (red dark, light red, yellow, and white) irradiated with 60 Gy of Y -radiation were stored for 6 months without sprouting, whereas 34.3% of the unirradiated onions developed sprouts [63].
Insect disinfection in cereals. Radiation is used for destroying insects in cereals, flours, and products made from them, since insects may damage some of these products. Insects can also serve as vectors for delivering pathogenic bacteria and parasites into food [58]. Radiation in the range of 100-1000 Gy is effective for inactivating insects in cereals [56, 64, 65]. Molds can be eliminated from cereals and grains by low dose irradiation. Doses > 1 kGy affect the quality of cereal grains and decrease flour viscosity. Also, they destroy starch and gluten, thus reducing the firmness, stickiness, and bulkiness of the dough [66].
Fruits and vegetables. Irradiation technology gives consumers access to the original taste, nutrients, appearance, and texture of fresh fruits and vegetables for extended periods of time. Radiation doses ˂ 1 kGy are recommended for insect disinfection of fruits and vegetables, as well as to delay their ripening and senescence and inhibit sprouting [17]. The radiation doses needed to extend shelf life and enhance the quality of fruits and vegetables depend on their maturity, cultivar, and pre- and post-harvest handling [67]. Lower doses (0.2-0.4 kGy) can increase the shelf life of fruits, while higher doses can lead to fruit decay [68]. The maximum irradiation dose for fresh fruit and vegetable disinfection and preservation must be < 1 kGy to receive approval from the United States Food and Drug Administration (FDA). Within the selected irradiation range, the sensitivity of the crop should be determined to enhance its quality without causing changes to its chemical and sensory properties [69].
The optimum dose for extending the shelf life of banana and plantain is within 150-300 Gy. At 500 Gy of irradiation, banana and plantain can develop a brown skin or gray scald due to increased polyphenol oxidase activity in the peels. Tissue damage can also be observed. Matured banana at harvest can tolerate quarantine radiation compared with less matured banana which develops peel injury after irradiation at 800 Gy [69]. Irradiation of fully matured green mangoes at 250–750 Gy can delay ripening. Tommy Atkins mangoes can be stored for 21 days at 12°C when exposed to radiation at ≤ 1000 Gy, while higher doses of ≥ 1500 Gy can result in fruit softening, peel scald, and flesh pitting [70-72].
Fish and meat products. The use of radiation in preserving fish can stabilize its market value and reduce wastage to ensure effective distribution of sea foods to the consumers [73]. Defrosted fish is contaminated with a lot of microorganisms, some of which move from the fish’s gastrointestinal tract into the muscle tissue causing spoilage. The shelf life of fish is relatively short due to the action of spoilage microorganisms and enzymes. This contributes to fermentation and breakdown of fish proteins into free amino acids, destroying the sensory properties of the fish. Ionizing radiation is a promising technology that can be employed to increase the shelf life of defrosted fish by inactivating or killing pathogens and spoilage microorganisms [74]. The treatment of fish fillets with radiation (1-4 kGy) is more effective than traditional preservation methods because radiation destroys microorganisms without changing the physicochemical properties of the fish [75]. However, doses > 4.5 kGy can cause oxidative damage and destroy vitamins, for example, thiamine (B1) [74]. Canned fish can be treated with low doses of irradiation after autoclaving to achieve good sterilization of the product. Exposure of cured fish to bacteria, fungi, and insects leads to its spoilage, so irradiation can be employed to reduce or eliminate these organisms in the product [73].
Meat is a perishable food product and the consumption of contaminated meat or meat products causes food-borne diseases. The most common pathogens that cause food-related outbreaks are C. perfringens, Salmonella, Shiga-toxigenic, Listeria monocytogenes, Clostridium botulinum, E. coli, Staphylococcus aureus, and B. cereus [76, 77]. Ultraviolet radiation (non-ionizing radiation) between 100-400 nm can be used to kill pathogenic microorganisms. Irradiation of fresh meat and meat products is considered a healthy and effective way to extend shelf life. Irradiation doses of 50 kGy or above can affect the meat color because of chemical changes caused to iron in myoglobin, while low doses (1-10 kGy) produce fewer changes in color, odor, and flavor [78]. Several studies confirmed the use of radiation to process meat products like ham, bacon, sausages, and beef burgers. Irradiation doses of 1-4 kGy can inactivate 90% of spoilage microorganisms in these products [79].
Dairy products. Milk and milk products form an important component in the food chain. Liquid, powdered, and concentrated milk is used in the food industry as a raw material for making different products. Consumers use milk for cooking and making beverages. Milk is susceptible to microbial contamination from cow’s udder, as well as at various stages of production, processing, and distribution [80]. Pathogenic microorganisms, such as L. monocytogenes, S. aureus, and Salmonella species, can contaminate milk and cause food-borne illnesses [81]. Heat pasteurization and sterilization are effectively used to decrease the microbial load. However, psychotropic bacterial species can continue to grow in milk at low temperatures and this is a major concern for the dairy industry [82]. Irradiation can be explored as an alternative technology to reduce or destroy microorganisms in raw milk before using it for the preparation of dairy products. The practical application of radiation to dairy products is relatively low because most pathogens are effectively eliminated by heat pasteurization. Also, some studies report off-flavors in irradiated dairy products [80, 83].
Several studies have evaluated the suitability of using irradiation to preserve dairy products such as cheese and ice cream. They recommended packaging the products before irradiation treatment in other to kill post-processing microbial contaminants [80]. Prior to cheese production, milk is pasteurized to reduce the microbial load, but this does not guarantee absolute microbial safety because the chances of post-pasteurization contamination are still high. Therefore, there is a need for exploring other non-thermal sterilization methods, such as irradiation technologies, to inactivate microorganisms present in the final product even after packaging [84]. Pseudomonas spp. and Enterobacteriaceae are the main organisms responsible for cheese spoilage [85]. A study by Lacivita et al. showed that X-rays at 2 and 3 kGy inactivated these species and extended the shelf life of Fiordilatte cheese for more than 40 days, while the unirradiated cheese remained unacceptable for about 10 days [83]. Food irradiation is safe and it has been approved in many countries. It can be applied to cheese to extend shelf life and maintain its nutritional and sensory properties [84].
Advantages and disadvantages of food irradiation. Advantages. Exposure of food to ionizing radiation aims to reduce microbial load by destroying microorganisms and inactivating enzymes present in food to prevent its spoilage. Radiation also prevents gametes of parasitic insects from reproducing, resulting in various food preservation outcomes [86]. Perishable fruits (bananas, pears, mangoes) can be irradiated to improve their shelf life. As a result, they can be delivered to consumers fresh and in a timely manner [87]. Pathogenic microorganisms, such as E. coli, Campylobacter, Listeria, Salmonella, Shigella, and Staphylococcus, can be present in many foods. These organisms are responsible for different kinds of food-borne diseases. Irradiation offers a positive way of eliminating these pathogenic organisms, especially in foods where thermal inactivation is inapplicable [87].
Disadvantages. Irradiation also affects the nutritional and sensory properties of foods, just like any other food processing method. While low and medium doses of irradiation do not affect the nutritional content, doses > 10 kGy can degrade nutrients, as seen in thermal food processing methods such as cooking and pasteurization. Vitamins are the most affected components during food irradiation treatment [13]. Ionizing radiation causes chemical changes in food through radiolysis. Certain parameters, such as absorbed dose, presence or absence of oxygen, dose rate and temperature, affect the chemical reactions or transformations induced in food products. Ionizing radiation at 10 kGy does not usually have any effect on micronutrients (water and fat-soluble vitamins) or macronutrients (carbohydrates, proteins, and lipids) in food. However, at doses > 10 kGy, macromolecules, such as carbohydrates, are decomposed and lipids become rancid [88, 89].
Direct energy absorption by irradiated foods can cause chemical changes through radiolysis effects. Irradiation causes water molecules to lose an electron giving rise to OH+, which then reacts with other water molecules to produce hydrogen peroxide (H2O2), hydroxyl radicals (OH•), molecular hydrogen, and oxygen. Since these molecules are free radicals, they cause oxidation and reduction reactions in food products [90, 91]. The effect of radiation on proteins depends on the protein structure, physical status, and amino acid content. The changes which can be induced in proteins include aggregation, dissociation, crosslinking, and oxidation [92]. For instance, y-irradiation of hazelnuts at 10 kGy caused denaturation and protein aggregation [93]. The use of high radiation doses for processing muscle foods, such as meat and meat products, leads to the formation of free radicals, as well as lipid and protein oxidation. This causes biochemical and physicochemical changes that affect the sensory and nutritional properties of the product [79].
CONCLUSION
Food spoilage and food-borne illnesses are caused by microorganisms which contaminate food during harvesting, manufacturing, and distribution. These microorganisms also reduce the shelf life of many processed and non-processed food products. The adoption of irradiation technologies to inactivate food spoilage microorganisms is gaining popularity among many food industries across the world. Food irradiation does not have much effect on the nutritional and chemical compositions of food. Thus, it is considered a good alternative to thermal processing methods. Radiation can be used to extend the shelf life of different kinds of foods, such as fruits, meat, fish, spices, tubers, bulbs, and many others.
Contribution
The authors were equally involved in writing the manuscript and are equally responsible for plagiarism.CONFLICTS OF INTEREST
The author declares that there is no conflict of interests regarding the publication of this article.ACKNOWLEDGEMENTS
The research funding from the Ministry of Science and Higher Education of the Russian Federation (Ural Federal University Program of Development within the Priority-2030 Program) is gratefully acknowledged.FUNDING
The research was performed on the Ministry of Science and Higher Education of the Russian Federation (Minobrnauki) (Ural Federal University Program of Development within the Priority-2030 Program) is gratefully acknowledged.REFERENCES
- Zhu J, Sun X, Zhang Z-D, Tang Q-Y, Gu M-Y, Zhang L-J, et al. Effect of ionizing radiation on the bacterial and fungal endophytes of the halophytic plant Kalidium schrenkianum. Microorganisms. 2021;9(5). https://doi.org/10.3390/microorganisms9051050
- Confalonieri F, Sommer S. Bacterial and archaeal resistance to ionizing radiation. Journal of Physics: Conference Series. 2011;261. https://doi.org/10.1088/1742-6596/261/1/012005
- Close DM, Nelson WH, Bernhard WA. DNA damage by the direct effect of ionizing radiation: Products produced by two sequential one-electron oxidations. Journal of Physical Chemistry A. 2013;117(47):12608–12615. https://doi.org/10.1021/jp4084844
- Rich T, Allen RL, Wyllie AH. Defying death after DNA damage. Nature. 2000;407:777–783. https://doi.org/10.1038/35037717
- Hoeijmakers JHJ. Genome maintenance mechanisms for preventing cancer. Nature. 2001;411:366–374. https://doi.org/10.1038/35077232
- Azzam EI, Jay-Gerin J-P, Pain D. Ionizing radiation-induced metabolic oxidative stress and prolonged cell injury. Cancer Letters. 2012;327:1–2:48–60. https://doi.org/10.1016/j.canlet.2011.12.012
- Madian AG, Regnier FE. Proteomic identification of carbonylated proteins and their oxidation sites. Journal of Proteome Research. 2010;9(8):3766–3780. https://doi.org/10.1021/pr1002609
- Maisonneuve E, Ducret A, Khoueiry P, Lignon S, Longhi S, Talla E, et al. Rules governing selective protein carbonylation. PLoS One. 2009;4(10). https://doi.org/10.1371/journal.pone.0007269
- Sukharev SA, Pleshakova OV, Moshnikova AB, Sadovnikov VB, Gaziev AI. Age- and radiation-dependent changes in carbonyl content, susceptibility to proteolysis, and antigenicity of soluble rat liver proteins. Comparative Biochemistry and Physiology Part B: Biochemistry and Molecular Biology. 1997;116(3):333–338. https://doi.org/10.1016/S0305-0491(96)00232-5
- Jung K-W, Lim S, Bahn Y-S. Microbial radiation-resistance mechanisms. Journal of Microbiology. 2017;55:499–507. https://doi.org/10.1007/s12275-017-7242-5
- Bisht B, Bhatnagar P, Gururani P, Kumar V, Tomar MS, Sinhmar R, et al. Food irradiation: Effect of ionizing and non-ionizing radiations on preservation of fruits and vegetables – a review. Trends in Food Science and Technology. 2021;114:327–385. https://doi.org/10.1016/j.tifs.2021.06.002
- Wang C-Y, Huang H-W, Hsu C-P, Yang BB. Recent advances in food processing using high hydrostatic pressure technology. Critical Reviews in Food Science and Nutrition. 2016;56(4):527–540. https://doi.org/10.1080/10408398.2012.745479
- Akhila PP, Sunooj KV, Aaliya B, Navaf M, Sudheesh C, Sabu S, et al. Application of electromagnetic radiations for decontamination of fungi and mycotoxins in food products: A comprehensive review. Trends in Food Science and Technology. 2021;114:399–409. https://doi.org/10.1016/j.tifs.2021.06.013
- Alqadi MK, Alzoubi FY, Jaber MA. Assessment of radon gas using passive dosimeter in Amman and Al-Rusaifa cities, Jordan. International Journal of Radiation Research. 2016;14(4):367–371. https://doi.org/10.18869/acadpub.ijrr.14.4.367
- Rafique M. Cesium-137 activity concentrations in soil and brick samples of Mirpur, Azad Kashmir; Pakistan. International Journal of Radiation Research. 2014;12(1):39–46.
- Agbaka JI, Ibrahim AN. Irradiation: Utilization, advances, safety, acceptance, future trends, and a means to enhance food security. Advances in Applied Science Research. 2020;11(3).
- Sharma P, Sharma SR, Mittal TC. Effects and application of ionizing radiation on fruits and vegetables: A review. Journal of Agricultural Engineering. 2020;57(2):97–126.
- Fernández Zenoff V, Siñeriz F, Farías ME. Diverse responses to UV-B radiation and repair mechanisms of bacteria isolated from high-altitude aquatic environments. Applied and Environmental Microbiology. 2006;72(12). https://doi.org/10.1128/AEM.01333-06
- Takeshita K, Shibato J, Sameshima T, Fukunaga S, Isobe S, Arihara K, et al. Damage of yeast cells induced by pulsed light irradiation. International Journal of Food Microbiology. 2003;85(1–2):151–158. https://doi.org/10.1016/S0168-1605(02)00509-3
- Krisko A, Radman M. Protein damage and death by radiation in Escherichia coli and Deinococcus radiodurans. Proceedings of the National Academy of Sciences. 2010;107(32):14373–14377. https://doi.org/10.1073/pnas.1009312107
- Blasius M, Hübscher U, Sommer S. Deinococcus radiodurans: What belongs to the survival kit? Critical Reviews in Biochemistry and Molecular Biology. 2008;43(3):221–238. https://doi.org/10.1080/10409230802122274
- Daly MJ. A new perspective on radiation resistance based on Deinococcus radiodurans. Nature Reviews Microbiology. 2009;7:237–245. https://doi.org/10.1038/nrmicro2073
- Sghaier H, Ghedira K, Benkahla A, Barkallah I. Basal DNA repair machinery is subject to positive selection in ionizing-radiation-resistant bacteria. BMC Genomics. 2008;9. https://doi.org/10.1186/1471-2164-9-297
- Ginoza W. The effects of ionizing radiation on nucleic acids of bacteriophages and bacterial cells. Annual Review of Microbiology. 1967;21:325–368. https://doi.org/10.1146/annurev.mi.21.100167.001545
- Riffo B, Henríquez C, Chávez R, Peña R, Sangorrín M, Gil-Duran C, et al. Nonionizing electromagnetic field: A promising alternative for growing control yeast. Journal of Fungi. 2021;7(4). https://doi.org/10.3390/jof7040281
- Ward JF. DNA damage produced by ionizing radiation in mammalian cells: Identities, mechanisms of formation, and reparability. 1988;35:95–125. https://doi.org/10.1016/S0079-6603(08)60611-X
- Hafer K, Rivina L, Schiestl RH. Cell cycle dependence of ionizing radiation-Induced DNA deletions and antioxidant radioprotection in Saccharomyces cerevisiae. Radiation Research. 2009;173(6):802–808. https://doi.org/10.1667/RR1661.1
- Raju MR, Gnanapurani M, Stackler B, Madhvanath U, Howard J, Lyman JT, et al. Influence of linear energy transfer on the radioresistance of budding haploid yeast cells. Radiation Research. 1972;51(2):310–317. https://doi.org/10.2307/3573612
- de Langguth EN, Beam CA. Repair mechanisms and cell cycle dependent variations in x-ray sensitivity of diploid yeast. Radiation Research. 1973;53(2):226–234. https://doi.org/10.2307/3573527
- Beam CA, Mortimer RK, Wolfe RG, Tobias CA. The relation of radioresistance to budding in Saccharomyces cerevisiae. Archives of Biochemistry and Biophysics. 1954;49(1):110–122. https://doi.org/10.1016/0003-9861(54)90172-1
- Coleine C, Stajich JE, Selbmann L. Fungi are key players in extreme ecosystems. Trends in Ecology and Evolution. 2022;37(6):517–528. https://doi.org/10.1016/j.tree.2022.02.002
- Zhdanova NN, Tugay T, Dighton J, Zheltonozhsky V, Mcdermott P. Ionizing radiation attracts soil fungi. Mycological Research. 2004;108(9):1089–1096. https://doi.org/10.1017/S0953756204000966
- Vember VV, Zhdanova NN. Peculiarities of linear growth of the melanin-containing fungi Cladosporium sphaerospermum Penz. and Alternaria alternata (Fr.) Keissler. Mikrobiolohichnyĭ Zhurnal. 2001;63(3):3–12.
- Dadachova E, Bryan RA, Huang X, Moadel T, Schweitzer AD, Aisen P, et al. Ionizing radiation changes the electronic properties of melanin and enhances the growth of melanized fungi. PLoS One. 2007;2(5). https://doi.org/10.1371/journal.pone.0000457
- Nosanchuk JD, Casadevall A. The contribution of melanin to microbial pathogenesis. Cellular Microbiology. 2003;5(4):203–223. https://doi.org/10.1046/j.1462-5814.2003.00268.x
- Dadachova E, Bryan RA, Howell RC, Schweitzer AD, Aisen P, Nosanchuk JD, et al. The radioprotective properties of fungal melanin are a function of its chemical composition, stable radical presence and spatial arrangement. Pigment Cell and Melanoma Research. 2008;21(2):192–199. https://doi.org/10.1111/j.1755-148X.2007.00430.x
- Jung K-W, Yang D-H, Kim M-K, Seo HS, Lim S, Bahn Y-S. Unraveling fungal radiation resistance regulatory networks through the genome-wide transcriptome and genetic analyses of Cryptococcus neoformans. mBio. 2016;7(6). https://doi.org/10.1128/mBio.01483-16
- Watson A, Mata J, Bähler J, Carr A, Humphrey T. Global gene expression responses of fission yeast to ionizing Radiation. Molecular Biology of the Cell. 2004;15(2):851–860. https://doi.org/10.1091/mbc.e03-08-0569
- Gasch AP, Huang M, Metzner S, Botstein D, Elledge SJ, Brown PO. Genomic expression responses to DNA-damaging agents and the regulatory role of the yeast ATR homolog Mec1p. Molecular Biology of the Cell. 2001;12(10):2987–3003. https://doi.org/10.1091/mbc.12.10.2987
- Isemberlinova AA, Egorov IS, Nuzhnyh SA, Poloskov AV, Pokrovskaya EA, Vertinskiy AV, et al. The pulsed X-ray treatment of wheat against pathogenic fungi. Nuclear Instruments and Methods in Physics Research Section B: Beam Interactions with Materials and Atoms. 2021;503:75–78. https://doi.org/10.1016/j.nimb.2021.07.011
- Munir MT, Federighi M. Control of foodborne biological hazards by ionizing radiations. Foods. 2020;9(7). https://doi.org/10.3390/foods9070878
- Nair PM, Sharma A. Food irradiation. In: Knoerzer K, Muthukumarappan K, editors. Innovative food processing technologies. A comprehensive review. Elsevier; 2016. pp. 19–29. https://doi.org/10.1016/B978-0-12-815781-7.02950-4
- Pathak B, Omre PK, Bisht B, Saini D. Effect of thermal and non-thermal processing methods on food allergens. Progressive Research – An International Journal. 2018;13(4):314–319.
- Prakash A, Ornelas-Paz JJ. Irradiation of fruits and vegetables. In: Yahia EM, editor. Postharvest technology of perishable horticultural commodities. Duxford: Woodhead Publishing; 2019. pp. 563–589. https://doi.org/10.1016/B978-0-12-813276-0.00017-1
- Barkai-Golan R, Follett PA. Sprout inhibition of tubers, bulbs, and roots by ionizing radiation. In: Barkai-Golan R, Follett PA, editors. Irradiation for quality improvement, microbial safety and phytosanitation of fresh produce. Cambridge: Academic Press; 2017. pp. 47–53. https://doi.org/10.1016/B978-0-12-811025-6.00005-7
- Bytesnikova Z, Adam V, Richtera L. Graphene oxide as a novel tool for mycotoxin removal. Food Control. 2021;121. https://doi.org/10.1016/j.foodcont.2020.107611
- Alshannaq A, Yu J-H. Occurrence, toxicity, and analysis of major mycotoxins in food. International Journal of Environmental Research and Public Health. 2017;14(6). https://doi.org/10.3390/ijerph14060632
- Roohi R, Hashemi SMB, Mousavi Khaneghah A. Kinetics and thermodynamic modelling of the aflatoxins decontamination: A review. International Journal of Food Science and Technology. 2020;55(12):3525–3532. https://doi.org/10.1111/ijfs.14689
- Mokhtarian M, Tavakolipour H, Bagheri F, Fernandes Oliveira CA, Corassin CH, Khaneghah AM. Aflatoxin B1 in the Iranian pistachio nut and decontamination methods: A systematic review. Quality Assurance and Safety of Crops and Foods. 2020;12(4):15–25. https://doi.org/10.15586/qas.v12i4.784
- de Souza C, Mousavi Khaneghah A, Fernandes Oliveira CA. The occurrence of aflatoxin M1 in industrial and traditional fermented milk: A systematic review study. Italian Journal of Food Science. 2021;33(SP1):12–23. https://doi.org/10.15586/ijfs.v33iSP1.1982
- Barba FJ, Koubaa M, do Prado-Silva L, Orlien V, de Souza Sant’Ana A. Mild processing applied to the inactivation of the main foodborne bacterial pathogens: A review. Trends in Food Science and Technology. 2017;66:20–35. https://doi.org/10.1016/j.tifs.2017.05.011
- Koca N, Urgu M, Saatli TE. Ultraviolet light applications in dairy processing. In: Koca N, editor. Technological approaches for novel applications in dairy processing. IntechOpen; 2018. https://doi.org/10.5772/intechopen.74291
- Moreno-Vilet L, Hernández-Hernández HM, Villanueva-Rodríguez SJ. Current status of emerging food processing technologies in Latin America: Novel thermal processing. Innovative Food Science and Emerging Technologies. 2018;50:196–206. https://doi.org/10.1016/j.ifset.2018.06.013
- Dogan Halkman HB, Yücel PK, Halkman AK. Non-thermal processing. Microwave. In: Batt CA, Tortorello ML, editors. Encyclopedia of food microbiology. Reference work. Academic Press; 2014. pp. 962–965. https://doi.org/10.1016/B978-0-12-384730-0.00400-6
- McKeen L. Introduction to food irradiation and medical sterilization. In: McKeen L, editor. The effect of sterilization on plastics and elastomers. Elsevier; 2018. pp. 1–40. https://doi.org/10.1016/B978-0-12-814511-1.00001-9
- Singh R, Singh A. Applications of food irradiation technology. Defence Life Science Journal. 2020;5(1):54–62. https://doi.org/10.14429/dlsj.5.14398
- Singh R, Singh A. Food irradiation: An established food processing technology for food safety and security. Defence Life Science Journal. 2019;4(4):206–213. https://doi.org/10.14429/dlsj.4.14397
- Mostafavi HA, Mirmajlessi SM, Fathollahi H. The potential of food irradiation: benefits and limitations. In: Eissa A, editor. Trends in vital food and control engineering. IntechOpen; 2012. pp. 43–68. https://doi.org/10.5772/34520
- Thanushree MP, Sailendri D, Yoha KS, Moses JA, Anandharamakrishnan C. Mycotoxin contamination in food: An exposition on spices. Trends in Food Science and Technology. 2019;93:69–80. https://doi.org/10.1016/j.tifs.2019.08.010
- Koopmans M, Duizer E. Foodborne viruses: an emerging problem. International Journal of Food Microbiology. 2004;90(1):23–41. https://doi.org/10.1016/S0168-1605(03)00169-7
- Singh A, Rao SR, Singh R, Chacharkar MP. Identification and dose estimation of irradiated onions by chromosomal studies. Journal of Food Science and Technology. 1998;35(1):47–50.
- Benkeblia N, Varoquaux P, Gouble B, Selselet- Attou G. Respiratory parameters of onion bulbs (Allium cepa) during storage. Effects of ionising radiation and temperature. Journal of the Science of Food and Agriculture. 2000;80(12):1772–1778. https://doi.org/10.1002/1097-0010(20000915)80:12<1772::AID-JSFA700>3.0.CO;2-5
- Tripathi PC, Sankar V, Mahajan VM, Lawande KE. Response of gamma irradiation on post harvest losses in some onion varieties. Indian Journal of Horticulture. 2011;68(4):556–560.
- Boshra SA, Mikhaiel AA. Effect of gamma irradiation on pupal stage of Ephestia calidella (Guenée). Journal of Stored Products Research. 2006;42(4):457–467. https://doi.org/10.1016/j.jspr.2005.09.002
- Azelmat K, Sayah F, Mouhib M, Ghailani N, ElGarrouj D. Effects of gamma irradiation on fourth-instar Plodia interpunctella (Hübner) (Lepidoptera: Pyralidae). Journal of Stored Products Research. 2005;41(4):423–431. https://doi.org/10.1016/j.jspr.2004.05.003
- Erkmen O, Bozoglu TF. Food preservation by irradiation. In: Erkmen O, Bozoglu TF, editors. Food microbiology: Principles into practice. Wiley; 2016. pp. 106–126. https://doi.org/10.1002/9781119237860.ch32
- Prakash A. Particular applications of food irradiation on fresh produce. Radiation Physics and Chemistry. 2016;129:50–52. https://doi.org/10.1016/j.radphyschem.2016.07.017
- Zhang K, Deng Y, Fu H, Weng Q. Effects of Co-60 gamma-irradiation and refrigerated storage on the quality of Shatang mandarin. Food Science and Human Wellness. 2014;3(1):9–15. https://doi.org/10.1016/j.fshw.2014.01.002
- Wall MM. Phytosanitary irradiation and fresh fruit quality: Cultivar and maturity effects. Stewart Postharvest Review. 2015;11(3):1–6. https://doi.org/10.2212/spr.2015.3.6
- Thomas P. Irradiation of fruits and vegetables. In: Molins RA, editor. Food irradiation: Principles and applications. New York: John Wiley & Sons; 2001. pp. 213–240.
- Reyes LF, Cisneros-Zevallos L. Electron-beam ionizing radiation stress effects on mango fruit (Mangifera indica L.) antioxidant constituents before and during postharvest storage. Journal of Agricultural and Food Chemistry. 2007;55(15):6132–6139. https://doi.org/10.1021/jf0635661
- Moreno M, Castell-Perez ME, Gomes C, Da Silva PF, Moreira RG. Effects of electron beam irradiation on physical, textural, and microstructural properties of “Tommy Atkins” mangoes (Mangifera indica L.). Journal of Food Science. 2006;71(2):E80–E86. https://doi.org/10.1111/j.1365-2621.2006.tb08900.x
- Vala RB, Vadher KH, Pampaniya NA, Parmar AM, Joshi A, Pushp A. Seafood irradiation – technology and application. International Journal of Advanced Research. 2016;4(6):132–136. https://doi.org/10.21474/IJAR01/625
- Timakova RT, Tikhonov SL, Tikhonova NV, Shikhalev SV. Determining the dose of radiation and radurisation effects on the antioxidant activity of fish and the thermophysical characteristics of fish muscle tissue. Foods. 2019;8(4). https://doi.org/10.3390/foods8040130
- Mohamed WS, El-Mossalami EI, Nosier SM. Evaluation of sanitary status of imported frozen fish fillets and its improvement by γ radiation. Journal of Radiation Research and Applied Sciences. 2009;2:921–931.
- Omer MK, Álvarez-Ordoñez A, Prieto M, Skjerve E, Asehun T, Alvseike OA. A systematic review of bacterial foodborne outbreaks related to red meat and meat products. Foodborne Pathogens and Disease. 2018;15(10):598–611. https://doi.org/10.1089/fpd.2017.2393
- Surveillance for foodborne disease outbreaks United States, 2017: Annual report. Atlanta: U.S. Department of Health and Human Services; 2019.
- Rebezov M, Chughtai MFJ, Mehmood T, Khaliq A, Tanweer S, Semenova A, et al. Novel techniques for microbiological safety in meat and fish industries. Applied Sciences. 2022;12(1). https://doi.org/10.3390/app12010319
- Jayathilakan K, Sultana K, Pandey MC. Radiation processing: An emerging preservation technique for meat and meat products. Defence Life Science Journal. 2017;2(2):133–141. https://doi.org/10.14429/dlsj.2.11368
- Odueke OB, Farag KW, Baines RN, Chadd SA. Irradiation applications in dairy products: A review. Food and Bioprocess Technology. 2016;9:751–767. https://doi.org/10.1007/s11947-016-1709-y
- Yagoub SO, Awadalla NE, El Zubeir IEM. Incidence of some potential pathogens in raw milk in Khartoun North Sudan and their susceptibility to antimicrobial agents. Journal of Animal and Veterinary Advances. 2005;4(3):341–344.
- de Oliveira GB, Favarin L, Luchese RH, McIntosh D. Psychrotrophic bacteria in milk: How much do we really know? Brazilian Journal of Microbiology. 2015;46(2):313–321. https://doi.org/10.1590/S1517-838246220130963
- Lacivita V, Mentana A, Centonze D, Chiaravalle E, Zambrini VA, Conte A, et al. Study of X-Ray irradiation applied to fresh dairy cheese. LWT. 2019;103:186–191. https://doi.org/10.1016/j.lwt.2018.12.073
- Nyamakwere F, Esposito G, Dzama K, Gouws P, Rapisarda T, Belvedere G, et al. Application of gamma irradiation treatment on the physicochemical and microbiological quality of an artisanal hard cheese. Applied Sciences. 2022;12(6). https://doi.org/10.3390/app12063142
- Mastromatteo M, Conte A, Lucera A, Saccotelli MA, Buonocore GG, Zambrini AV, et al. Packaging solutions to prolong the shelf life of Fiordilatte cheese: Bio-based nanocomposite coating and modified atmosphere packaging. LWT – Food Science and Technology. 2015;60(1):230–237. https://doi.org/10.1016/j.lwt.2014.08.013
- Farkas J. Irradiation for better foods. Trends in Food Science and Technology. 2006;17(4):148–152. https://doi.org/10.1016/j.tifs.2005.12.003
- Pricaz M, Uta A-C. Gamma radiation for improvements in food industry, environmental quality and healthcare. Romanian Journal of Biophysics. 2015;25(2):143–162.
- Brewer MS. Irradiation effects on meat flavor: A review. Meat Science. 2009;81(1):1–14. https://doi.org/10.1016/j.meatsci.2008.07.011
- Miller RB. Electronic irradiation of foods: An introduction to the technology. New York: Springer; 2005. 296 p. https://doi.org/10.1007/0-387-28386-2
- Calucci L, Pinzino C, Zandomeneghi M, Capocchi A, Ghiringhelli S, Saviozzi F, et al. Effects of γ-irradiation on the free radical and antioxidant contents in nine aromatic herbs and spices. Journal of Agricultural and Food Chemistry. 2003;51(4):927–934. https://doi.org/10.1021/jf020739n
- Black JL, Jaczynski J. Effect of water activity on the inactivation kinetics of Escherichia coli O157:H7 by electron beam in ground beef, chicken breast meat, and trout fillets. International Journal of Food Science and Technology. 2008;43(4):579–586. https://doi.org/10.1111/j.1365-2621.2006.01480.x
- Mostafavi HA, Fathollahi H, Motamedi F, Mirmajlessi SM. Food irradiation: Applications, public acceptance and global trade. African Journal of Biotechnology. 2010;9(20):2826–2833.
- Dogan A, Siyakus G, Severcan F. FTIR spectroscopic characterization of irradiated hazelnut (Corylus avellana L.). Food Chemistry. 2007;100(3):1106–1114. https://doi.org/10.1016/j.foodchem.2005.11.017